“Gamma Synchrony” in First-Episode Schizophrenia: A Disorder of Temporal Connectivity?
Abstract
OBJECTIVE: There has been a convergence of models describing schizophrenia as a disconnection syndrome, with a focus on the temporal connectivity of neural activity. Synchronous gamma-band (40-Hz) activity has been implicated as a candidate mechanism for the binding of distributed neural activity. To the authors’ knowledge, this is the first study to investigate “gamma synchrony” in first-episode schizophrenia. METHOD: Forty medicated first-episode schizophrenia patients and 40 age- and sex-matched healthy comparison subjects participated in a conventional auditory oddball paradigm. Gamma synchrony, time-locked to target stimuli, was extracted from an ongoing EEG. The magnitude and latency of both early (gamma 1: –150 msec to 150 msec poststimulus) and late (gamma 2: 200 to 550 msec poststimulus) synchrony were analyzed with multiple analysis of variance. RESULTS: First-episode schizophrenia patients showed a decreased magnitude and delayed latency for global gamma 1 synchrony in relation to healthy comparison subjects. By contrast, there were no group differences in gamma 2 synchrony. CONCLUSIONS: These findings suggest that first-episode schizophrenia patients have a global decrease and delay of temporal connectivity of neural activity in early sensory response to task-relevant stimuli. This is consistent with cognitive evidence of perceptual integration deficits in this disorder and raises the possibility that a breakdown in the early synchrony of distributed neural networks is a marker for the onset of schizophrenia.
More than 90 years ago, Bleuler (1, p. 9) proposed the term “schizophrenia” to describe a disease process that produces a “splitting of the psychic functions.” More recently, the failure of a “single-lesion” model to account for the pathophysiology of schizophrenia has led to a renewed focus on “disconnection syndrome” models (2, 3). These models draw on recent neuroscientific findings and highlight a lack of structural and functional connectivity across multiple brain systems (4–6).
Cognitive models of schizophrenia are consistent with disconnection accounts. Common to these models is the proposal that schizophrenia represents a disturbed integration of incoming sensory input with regularities stored in memory (7–9). This lack of integration is reflected in deficits of perceptual organization (10), attention to relevant signals and inhibition of irrelevant signals (11, 12), and defective metacognitive processes (13). Braff (11) has attributed information-processing dysfunctions in schizophrenia to a miscoordination of distributed neural networks that would normally function in an integrated and time-linked manner.
Andreasen and colleagues (4, 5, 14, 15) coined the term “cognitive dysmetria” to emphasize the temporal dimension of neural disconnection in schizophrenia. It is proposed that a “disruption to fluid coordination of mental activity” at the “nanosecond” time scale impairs the ability to integrate and contextualize incoming sensory input in order to form an appropriate and adaptive response (15) (p. 911). Andreasen and colleagues hypothesized that a lack of connectivity in cortical-cerebellar-thalamal-cortical circuitry underlies cognitive dysmetria. Although the cortical-cerebellar-thalamal-cortical circuitry spatial dimension of cognitive dysmetria has been investigated (16–19), this is the first study to our knowledge to use a high-temporal-resolution measure to investigate distributed brain network integration in first-episode schizophrenia.
Synchronization (phase-locking) has been proposed as a mechanism for the integration, or “binding,” of activity in distributed neural networks (20, 21). In-phase, or synchronous, neuronal activity has been observed across species and across both microscopic and mesoscopic scales of recording with depth electrode and scalp recordings from EEGs (see reference 22 for a review). Notably, such synchronous activity has been associated with oscillations in the gamma frequency band (varying from 30 to 90 Hz, depending on the task), prompting the description of gamma-band activity as the “building block” of brain function (23). Synchronous gamma-band activity has been observed in healthy humans in response to sensory stimulation and a range of cognitive tasks (22), including both auditory and visual stimulation (24–26) and learned associations (27).
Using a measure that extracts in-phase, synchronous gamma-band activity across multiple distributed brain regions from EEGs, we demonstrated previously that the auditory oddball paradigm elicits peaks in narrow-band “gamma synchrony” of 39–41 Hz around stimulus onset (<150 msec poststimulus; gamma 1) for both target and background stimuli and between 250 and 550 msec poststimulus (gamma 2) for target stimuli only (25). These observations suggest that there is an early peak in gamma synchrony associated with preparatory/perceptual processing of sensory input and a later peak associated specifically with selective context processing of task-relevant signals.
Gamma synchrony provides a pertinent index of thalamal-cortical synchrony. In a numerical simulation of whole brain neurophysiology, Rennie et al. (28) demonstrated that gamma activity is enhanced with increased activity in the thalamal-cortical feedback loop. Jones (29) proposed that in schizophrenia, a failure of neuronal binding in the cortical-reticulal-thalamal-cortical loop is reflected in disturbed synchronization in the gamma frequency band. This disturbance is associated with an inability to focus attention on relevant stimuli in the face of irrelevant stimuli and to select appropriate responses. To date, studies have mainly focused on abnormalities in the amplitude (or power) of gamma activity in schizophrenia (30–33) rather than on the synchronization of gamma activity, which provides a more direct measure of neural binding.
Our group’s study of gamma synchrony in chronic schizophrenia revealed a lack of temporal connectivity across distributed networks, with decreased frontal (gamma 1 and 2) and left hemisphere gamma 1 synchrony but increased posterior gamma 2 synchrony to target stimuli, relative to healthy comparison subjects (34). These findings are consistent with a core disturbance of temporal connectivity (or “cognitive dysmetria”) across cortical-cerebellar-thalamal-cortical circuitry, which impairs the perceptual and cognitive processing of target stimuli.
The present study investigated gamma synchrony in first-episode schizophrenia. Given that the long-term effects of medication, illness chronicity, and institutionalization are minimized in these individuals, this study provided a first step in elucidating the role of temporal connectivity in the initial stages of schizophrenia (35, 36). We predicted that the gamma synchrony disturbances observed in chronic schizophrenia (34) would also be present in first-episode schizophrenia, consistent with the view that disturbances in the temporal connectivity of distributed cortical-cerebellar-thalamal-cortical circuitry are a marker of this disorder.
Method
Subjects
Forty first-episode schizophrenia patients (mean age=19.6 years, SD=3.2, range=14–26 years; 26 men and 14 women) who were recruited from the Western Sydney Psychosis Early Intervention and Recovery Service (an outpatient service) and 40 age- and sex-matched healthy comparison subjects (mean age=19.7 years, SD=3.9 years, range=14–29 years) participated in the study. The comparison subjects were matched within 1 year of age for all but three subjects, who were matched within 3 years of age. The healthy comparison subjects were also matched on intellectual functioning, estimated with the WAIS (37) and the Kaufman Brief Intelligence Test (38), within half a standard deviation (schizophrenia patients: mean=94.40, SD=10.45; healthy comparison subjects: mean=100.73, SD=9.11). Intelligence testing was undertaken before treatment in the schizophrenia patients and did not differ significantly from premorbid IQ estimates (assessed with the New Adult Reading Test [39]). First-episode schizophrenia patients were carefully selected for a confirmed diagnosis of schizophrenia from a larger pool of 65 first-episode patients, comprising additional diagnostic categories (bipolar disorder, major depressive disorder, brief psychotic disorder, and drug-induced psychosis). The diagnosis of schizophrenia was confirmed according to both DSM-IV and ICD-10 criteria, and diagnostic consensus was achieved by three psychiatrists independent of the study on the basis of semistructured interviews with the patient, the family, and case managers, as well as from detailed case notes. For both groups, exclusion criteria were left-handedness; a recent history of substance abuse, epilepsy, or other neurological disorders; and head injury, which were assessed with section M from the Composite International Diagnostic Interview (40) and the Westmead Hospital clinical information base (41).
Of the 40 first-episode patients, 36 were taking atypical neuroleptic medications (19 risperidone, 15 olanzapine, one clozapine, and one quetiapine), and four were unmedicated. The mean medication level (measured in chlorpromazine equivalents [42, 43]) was 256 mg (SD=227, range=0–667), and the mean treatment duration was 10.2 months (SD=8.1, range=0–23). Doses (in chlorpromazine equivalents) for individual medications were as follows: risperidone (mean=199, SD=170, range=33–667), olanzapine (mean=392.9, SD=252.7, range=67–667), clozapine (mean=333, SD=0), and quetiapine (mean=250, SD=0).
Symptom severity was assessed with the Positive and Negative Syndrome Scale (44), and severity was in the mild to moderate range as follows: positive symptoms (mean=17.1, SD=5.7, range=7–27), negative symptoms (mean=20.13, SD=6.0, range=9–35), and general psychopathology (mean=38.9, SD=6.7, range=25–50).
After receiving a complete description of the study, each subject (or parent/guardian) provided written informed consent in accordance with National Health and Medical Research Council ethical guidelines.
Apparatus and Stimuli
EEG data were acquired during an auditory oddball paradigm (40 target tones at 1500 Hz and 250 background tones at 1000 Hz). The tones (50 msec in duration, 10 msec of rise and fall time, and at 80 dB above the hearing threshold) were presented binaurally through stereo headphones at an interstimulus interval of one per 1.3 seconds. They were delivered pseudorandomly, with the constraint that there were no successive target stimuli.
EEG data were recorded from 19 scalp electrode sites, according to the international 10–20 system with an electrode cap (45). Linked earlobes served as a reference. Horizontal and vertical eye-movement potentials were recorded with two electrodes placed 1 cm lateral to the outer canthus of each eye and on the middle of supraorbital and infraorbital regions of the left eye, respectively. Impedance of all electrodes was less than 5 kΩ. A continuous acquisition system was employed with a 250-Hz digitization rate. Electro-oculogram (EOG) correction was carried out off-line (46).
Procedure
The subjects were seated in a sound- and light-attenuated room. They were instructed to ignore background tones and to press a reaction-time button to target tones with the first finger of each hand (to counterbalance for possible motor effects). Speed and accuracy were emphasized equally in the task instructions. To limit EOG contamination, the subjects were instructed to look at a small dot on a computer screen placed 60 cm in front of them during the task. All subjects were asked to refrain from smoking or drinking caffeine for 3 hours before the recording session. There were data for a minimum of 30 target responses for each subject for inclusion in the focal analyses after artefact rejection was applied. The schizophrenia and comparison groups did not differ in the number of correct target responses.
Data Analysis
A narrow gamma-band signal (37–41 Hz) was examined in line with our previous evidence that this frequency range contains the key aspects of information processing in the oddball paradigm (47). To minimize contamination of broad range EEG signals (i.e., electromyograph effects), all single trials were detrended by subtracting the line of best fit over 512 samples (sampling rate of 256 Hz) centered at the stimulus presentation. At each sample position (from –500 to 750 msec), a 64-sample Welch window was moved along sample by sample, and the phase of the gamma frequency component was computed by means of fast Fourier transformation. This procedure produced a time series of gamma phase from each electrode site, which enabled gamma synchrony to be time-locked to individual target trials.
To calculate gamma synchrony, the phase of the given electrode sites was computed with circular variance (48), yielding a singular estimate of the degree of phase locking at each time point across sites. The single-trial waveforms were then averaged over individual trials for which there was a correct response. For each averaged waveform, we scored the magnitude and the latency for the peak (or maximal) synchrony in both the gamma 1 (–150 to 150 msec poststimulus) and gamma 2 (200 to 550 msec poststimulus) windows.
Following the analysis procedure for our study of chronic schizophrenia (34), gamma 1 and gamma 2 synchrony (magnitude and latency) were calculated in anterior or frontal (Fp1/2, F3/4, F7/8, and Fz) and posterior (T5/6, P3/4, Pz, and O1/2) regions to examine functional integration in anterior-posterior networks and left (Fp1, F3, F7, T3, T5, C3, P3, and O1) and right (Fp2, F4, F8, T4, T6, C4, P4, and O2) hemisphere synchrony to examine laterality and intrahemispheric integrative function. The magnitude and latency of gamma 1 and 2 synchrony were analyzed with mixed-model multiple analysis of variance (MANOVA), with the between-subjects variable of group (first-episode schizophrenia patients versus comparison subjects) and within-subjects factor of region (anterior versus posterior or laterality) used to examine topographical effects. MANOVA group main effects were examined in terms of group differences in global gamma synchrony (across all sites used in topographical analyses). The possible confounding effects of chlorpromazine medication dose on group differences was examined with analysis of covariance (MANCOVA).
Results
Reaction Time Data
Consistent with previous studies (30, 32, 34), the schizophrenia patients had a significantly slower reaction time to target stimuli than the healthy comparison subjects (comparison subjects: mean=309.6 msec, SD=41.4, range=225–466; schizophrenia patients: mean=384.6 msec, SD=106.2, range=239–702) (t=4.16, df=78, p<0.0001).
Global Gamma Synchrony
Table 1 presents descriptive data for global gamma synchrony for both the schizophrenia and healthy comparison groups. The first-episode schizophrenia patients showed a significantly lower magnitude of global gamma 1 (F=18.78, df=1, 78, p≤0.0005) in relation to the healthy comparison subjects. The patient group also showed a significant delay in the latency of global gamma 1 synchrony (F=5.85, df=1, 78, p<0.02) relative to the comparison subjects. By contrast, there were no significant group differences in the magnitude (F=0.42, df=1, 78, p=0.52) or latency (F=0.70, df=1, 78, p=0.41) of gamma 2 synchrony in relation to the healthy comparison subjects.
Anterior Versus Posterior Gamma Synchrony
MANOVA revealed a significant interaction between group and the anterior/posterior factor for the magnitude of gamma 1 (F=10.81, df=1, 78, p=0.002) but not gamma 2 (F=1.61, df=1, 78, p=0.21) synchrony. Although the healthy comparison subjects showed significantly higher gamma 1 synchrony anteriorly than posteriorly (F=4.76, df=1, 78, p<0.04), this pattern was reversed in the first-episode schizophrenia patients, who showed significantly less anterior than posterior gamma 1 synchrony (F=6.09, df=1, 78, p<0.02). The first-episode schizophrenia patients also showed significantly less gamma 1 synchrony peak amplitude in relation to the healthy comparison subjects (F=26.68, df=1, 78, p≤0.0005) (Figure 1). There were no significant interactions in the latency of gamma 1 (F=1.37, df=1, 78, p=0.25) or gamma 2 synchrony (F=0.84, df=1, 78, p=0.36). Figure 1 depicts the average waveform for gamma synchrony within the anterior (frontal) region for the first-episode schizophrenia and healthy comparison groups.
Left Versus Right Hemisphere Gamma Synchrony
There was no significant interaction between group and laterality for the magnitude of gamma 1 (F=0.08, df=1, 78, p=0.78) or gamma 2 (F=0.13, df=1, 78, p=0.72) synchrony. Similarly, there was no significant interaction for the latency of gamma 1 (F=0.52, df=1, 78, p=0.47) or gamma 2 (F=0.67, df=1, 78, p=0.42) synchrony.
Effect of Medication
MANCOVA indicated that medication level (in chlorpromazine equivalents) did not covary significantly with between-group differences in the magnitude of gamma 1 synchrony. However, the delay in global gamma 1 synchrony was reduced to borderline significance (F=3.91, df=1, 78, p=0.052) when the effect of medication level was taken into account. Reaction time did not covary significantly with any of the between-group findings.
Discussion
In this study, we used a high-temporal-resolution (millisecond time scale) measure, gamma synchrony, to examine disturbances of functional brain connectivity in first-episode schizophrenia patients versus healthy comparison subjects in response to task-relevant stimuli. The first-episode schizophrenia patients exhibited a decrease in early (gamma 1; –150 to 150 msec poststimulus) synchrony in relation to the healthy comparison subjects. By contrast, there were no impairments in synchrony during the later (200 to 550 msec poststimulus) period of processing. The lower levels of early synchronization of the schizophrenia patients were most apparent in the frontal region. Although the schizophrenia patients showed a gradient of reduced frontal relative to posterior synchrony, the healthy comparison subjects showed the opposite pattern of anterior-posterior synchrony. Although we observed a concomitant delay in early synchrony in the schizophrenia patients, this finding was due, in part, to the effects of neuroleptic medication.
Our findings for first-episode schizophrenia are consistent with models describing schizophrenia as a “disconnection syndrome” in which the precise temporal coordination (or functional connectivity) of distributed neural activity is disrupted (11, 14, 15). The observation that synchrony was reduced frontally in first-episode patients accords with our group’s previous finding in chronic schizophrenia (34), as well as preliminary evidence from a study of first-episode schizophrenia in relation to sex differences (49). The occurrence of this frontal impairment in the early expression of schizophrenia suggests that it is unlikely to be a consequence of chronicity. Rather, a lack of frontal synchronization may have a trait-like status in schizophrenia, suggesting its potential as a marker of this disorder.
The present findings of reduced early frontal synchrony in first-episode schizophrenia are consistent with the “hypofrontality” hypothesis of schizophrenia (50). Yet these findings suggest that rather than a reduction in volume or activity alone, there is a fundamental lack of functional connectivity in the frontal lobe. This proposal accords with the proposed mechanisms of gamma synchrony. Depth-recording evidence suggests that short-range gamma synchrony results from the mutual inhibition of γ-aminobutyric acid (GABA)-ergic interneurons and long-range synchrony from the precise in-phase synchronization of interneuron networks and pyramidal cells (51, 52). In postmortem studies, schizophrenia patients showed a selective decrease of 40% in the axon terminal density of chandelier neurons—GABA-ergic interneurons that synapse exclusively with pyramidal cells (53). The decrease in GABA-ergic binding in schizophrenia is most apparent in the frontal cortex (54) and is not explained by medication (53).
The decrease and delay of early gamma synchrony observed in first-episode schizophrenia is also consistent with cognitive models of a perceptual organization deficit in this disorder (10). This impaired ability to integrate stimulus components into object representations in schizophrenia is believed to occur within 200-msec poststimulus, overlapping the temporal window within which early gamma synchrony occurs. Such a disturbance might lead to an inability to discriminate relevant from irrelevant sensory input into consciousness (11, 12). Indeed, Clementz et al. (55) suggested that gamma-band abnormalities might account for schizophrenia reductions in P50 suppression, an index of sensory gating (11). Our finding that first-episode patients were delayed in identifying target stimuli (albeit accurately) may reflect the flow-on consequences of disruptions to early sensory integration. While reaction time to targets has been associated with gamma 2 synchrony in healthy subjects (48), the lack of gamma 2 disturbances in first-episode patients suggests that performance delays are not due to a breakdown in more controlled, contextual processing.
Although disturbed frontal connectivity has now been demonstrated in both first-episode and chronic schizophrenia, the stage of processing in which this disturbance occurs appears to differ between the early and chronic phases of this disorder. The lack of temporal connectivity in first-episode patients was associated with the early integration of sensory input only. By contrast, chronically ill patients show desynchronization during later contextual processing (gamma 2) as well as during early sensory integration (gamma 1), which is localized breakdown in the left hemisphere as well as the frontal cortex (30, 32, 34). Wright and Kydd (56) proposed the notion of an evolving pathophysiology in schizophrenia, with compensatory maladaptions unfolding in the pursuit of homeostasis. From this perspective, the reduction and delay in early synchrony in first-episode schizophrenia might represent an original state of neural disharmony resulting in sensory overload, with the more topographically distributed breakdown of later context-related synchrony arising as an attempted compensation with illness chronicity. Longitudinal investigation of gamma synchrony in schizophrenia would be important in testing this proposal and in discounting the alternative possibility that chronically ill schizophrenia patients represent a distinct pathophysiology.
Our observation that deficits in synchrony are present in the first episode of schizophrenia and may progress with chronicity is consistent with the notion that schizophrenia may be a “magnification” of variants in connectivity (57) along a continuum between “normality” and frank psychosis (58). The investigation of gamma synchrony in high-risk individuals might provide an understanding of the links between schizophrenia and normality, as well as earlier stages in the development of schizophrenia (11).
Peled (59) has suggested that different patterns of psychopathology within schizophrenia might emerge from different patterns of failed neural connectivity (or “multiple constraint organization”). Our group (34) found evidence of different patterns of gamma synchrony disturbances associated with psychomotor poverty, reality distortion, and disorganization syndromes in chronic schizophrenia. The investigation of relationships between symptom profiles and gamma synchrony disturbances in a first-episode sample might provide further understanding of the clinical implications of gamma synchrony disturbances.
Future studies are also warranted to clarify several other matters regarding disturbances of neural synchronization in first-episode schizophrenia. First, although medication dose did not account for disturbances in the magnitude of gamma synchrony in this study, the correlational design makes it difficult to explicitly disentangle the potential contribution of medication. Therefore, it would be important to confirm these findings of impaired synchronization in a direct comparison of medicated and unmedicated first-episode schizophrenia patients. Second, the employment of a variety of cognitive paradigms would also help to elucidate the relationship between gamma synchrony abnormalities and dysfunctions of sensory and information processing. It is possible that more complex tasks—and a greater cognitive load—would elicit gamma 2 as well as gamma 1 synchrony disturbances in first-episode schizophrenia patients. In extending this research to other cognitive tasks, the contribution of synchronization in other frequency bands and its functional significance should also be considered (60). Third, the relationship between disturbed gamma synchrony and constitutional characteristics such as gender warrants attention, given the gender bias in the incidence of schizophrenia and preliminary indications that gamma synchrony varies with gender (49). Fourth, it would be important to examine the specificity of these disturbances to the diagnosis of schizophrenia, particularly in relation to other psychotic disorders, given overlaps in symptom profiles (35, 61) and the nonspecificity of neuroleptic medications for psychotic symptoms (62). A comparison of schizophrenia and affective psychosis is currently underway.
The present study is the first to our knowledge to demonstrate disturbed temporal connectivity in first-episode schizophrenia with a gamma synchrony measure. The reduction and delay in early synchrony in the frontal cortex might represent a marker for the early manifestation of schizophrenia. The lower level of early frontal synchrony might represent a trait-like marker of schizophrenia that is consistent with neurobiological evidence of selective GABA-ergic deficits in the frontal cortex as well as cognitive evidence for an impairment in perceptual integration. Given that GABA-ergic mechanisms have been implicated in the action of antipsychotic medications and the generation of gamma synchrony, an understanding of gamma activity has the potential to provide a basis for improved interventions for schizophrenia.
![]() |
Received Dec. 9, 2003; revision received July 13, 2004; accepted July 19, 2004. From the Brain Dynamics Centre, Westmead Hospital; the Discipline of Psychological Medicine and the School of Psychology, University of Sydney, Sydney, N.S.W., Australia. Address reprint requests to Dr. Williams, The Brain Dynamics Centre, Acacia House, Westmead Hospital, Westmead, N.S.W., 2145 Australia; [email protected] (e-mail).
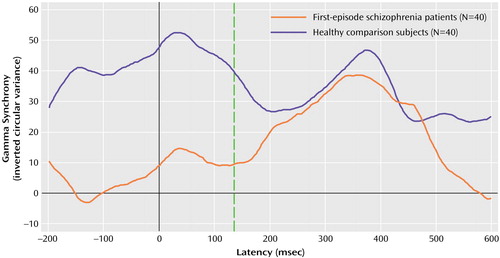
Figure 1. Group Average Gamma Synchrony Waveforms in the Brain’s Frontal Region for Healthy Comparison Subjects and First-Episode Schizophrenia Patientsa
aEarly gamma synchrony (around stimulus onset, broken vertical line) was significantly lower in schizophrenia patients than in healthy comparison subjects.
1. Bleuler E: Dementia Praecox or the Group of Schizophrenias (1911). Translated by Zinkin J. New York, International Universities Press, 1950Google Scholar
2. Friston KJ, Frith CD: Schizophrenia: a disconnection syndrome? Clin Neurosci 1995; 3:89–97Medline, Google Scholar
3. Friston KJ: Schizophrenia and the disconnection hypothesis. Acta Psychiatr Scand Suppl 1999; 395:68–79Crossref, Medline, Google Scholar
4. Andreasen NC: A unitary model of schizophrenia: Bleuler’s “fragmented phrene” as schizencephaly. Arch Gen Psychiatry 1998; 56:781–787Crossref, Google Scholar
5. Andreasen NC: Linking mind and brain in the study of mental illness: a project for a scientific psychopathology. Science 1997; 275:1586–1593Crossref, Medline, Google Scholar
6. Braff DL: Connecting the “dots” of brain dysfunction in schizophrenia: what does the picture look like? Arch Gen Psychiatry 1999; 56:791–793Crossref, Medline, Google Scholar
7. Gray JA: Integrating schizophrenia. Schizophr Bull 1998; 24:249–266Crossref, Medline, Google Scholar
8. Hemsley DR: A simple (or simplistic?) cognitive model for schizophrenia. Behav Res Ther 1993; 31:633–645Crossref, Medline, Google Scholar
9. Hemsley DR: Schizophrenia: a cognitive model and its implications for psychological intervention. Behav Modif 1996; 20:139–169Crossref, Medline, Google Scholar
10. Silverstein SM, Knight RA, Schwarzkopf SB, West LL, Osborn LM, Kamin D: Stimulus configuration and context effects in perceptual organization in schizophrenia. J Abnorm Psychol 1996; 105:410–420Crossref, Medline, Google Scholar
11. Braff DL: Information processing and attention dysfunctions in schizophrenia. Schizophr Bull 1993; 19:233–259Crossref, Medline, Google Scholar
12. Frith CD: Consciousness, information processing and schizophrenia. Br J Psychiatry 1979; 134:225–235Crossref, Medline, Google Scholar
13. Frith CD: The Cognitive Neuropsychology of Schizophrenia. Hove, UK, Lawrence Erlbaum Associates, 1992Google Scholar
14. Andreasen NC, Paradiso S, O’Leary DS: “Cognitive dysmetria” as an integrative theory of schizophrenia: a dysfunction in cortical-subcortical-cerebellar circuitry? Schizophr Bull 1998; 24:203–218Crossref, Medline, Google Scholar
15. Andreasen NC, Nopoulos P, O’Leary DS, Miller DD, Wassink T, Flaum M: Defining the phenotype of schizophrenia: cognitive dysmetria and its neural mechanisms. Biol Psychiatry 1999; 46:908–920Crossref, Medline, Google Scholar
16. Andreasen NC, O’Leary DS, Cizadlo T, Arndt S, Rezai K, Ponto LL, Watkins GL, Hichwa RD: Schizophrenia and cognitive dysmetria: a positron-emission tomography study of dysfunctional prefrontal-thalamic-cerebellar circuitry. Proc Natl Acad Sci USA 1996; 93:9985–9990Crossref, Medline, Google Scholar
17. Crespo-Facorro B, Paradiso S, Andreasen NC, O’Leary DS, Watkins GL, Boles Ponto LL, Hichwa RD: Recalling word lists reveals “cognitive dysmetria” in schizophrenia: a positron emission tomography study. Am J Psychiatry 1999; 156:386–392Abstract, Google Scholar
18. Wiser AK, Andreasen NC, O’Leary DS, Watkins GL, Boles Ponto LL, Hichwa RD: Dysfunctional cortical-cerebellar circuits cause “cognitive dysmetria” in schizophrenia. Neuroreport 1998; 9:1895–1899Crossref, Medline, Google Scholar
19. Volz H, Gaser C, Sauer H: Supporting evidence for the model of cognitive dysmetria in schizophrenia—a structural magnetic resonance imaging study using deformation-based morphometry. Schizophr Res 2000; 46:45–56Crossref, Medline, Google Scholar
20. Engel AK, Singer W: Temporal binding and the neural correlates of sensory awareness. Trends Cogn Sci 2001; 5:16–25Crossref, Medline, Google Scholar
21. Singer W, Gray CM: Visual feature integration and the temporal correlation hypothesis. Annu Rev Neurosci 1995; 18:555–586Crossref, Medline, Google Scholar
22. Lee KH, Williams LM, Breakspear M, Gordon E: Synchronous gamma activity: a review and contribution to an integrative neuroscience model of schizophrenia. Brain Res Brain Res Rev 2003; 41:57–78Crossref, Medline, Google Scholar
23. Basar-Eroglu C, Struber D, Schurmann M, Stadler M, Basar E: Gamma-band responses in the brain: a short review of psychophysiological correlates and functional significance. Int J Psychophysiol 1996; 24:101–112Crossref, Medline, Google Scholar
24. Bhattacharya J, Petsche H: Musicians and the gamma band: a secret affair? Neuroreport 2001; 12:371–374Crossref, Medline, Google Scholar
25. Haig AR, Gordon E, Wright JJ, Meares RA, Bahramali H: Synchronous cortical gamma-band activity in task-relevant cognition. Neuroreport 2000; 11:669–675Crossref, Medline, Google Scholar
26. Rodriguez E, George N, Lachaux JP, Martinerie J, Renault B, Varela FJ: Perception’s shadow: long-distance synchronization of human brain activity. Nature 1999; 397:430–433Crossref, Medline, Google Scholar
27. Miltner WH, Braun C, Arnold M, Witte H, Taub E: Coherence of gamma-band EEG activity as a basis for associative learning. Nature 1999; 397:434–436Crossref, Medline, Google Scholar
28. Rennie CJ, Wright JJ, Robinson PA: Mechanisms of cortical electrical activity and emergence of gamma rhythm. J Theor Biol 2000; 205:17–35Crossref, Medline, Google Scholar
29. Jones EG: Cortical development and thalamic pathology in schizophrenia. Schizophr Bull 1997; 23:483–501Crossref, Medline, Google Scholar
30. Haig AR, Gordon E, De Pascalis V, Meares RA, Bahramali H, Harris A: Gamma activity in schizophrenia: evidence of impaired network binding? Clin Neurophysiol 2000; 111:1461–1468Crossref, Medline, Google Scholar
31. Kissler J, Muller MM, Fehr T, Rockstroh B, Elbert T: MEG gamma band activity in schizophrenia patients and healthy subjects in a mental arithmetic task and at rest. Clin Neurophysiol 2000; 111:2079–2087Crossref, Medline, Google Scholar
32. Lee KH, Williams LM, Haig A, Goldberg E, Gordon E: An integration of 40 Hz Gamma and phasic arousal: novelty and routinization processing in schizophrenia. Clin Neurophysiol 2001; 112:1499–1507Crossref, Medline, Google Scholar
33. Slewa-Younan S, Gordon E, Williams L, Haig AR, Goldberg E: Sex differences, gamma activity and schizophrenia. Int J Neurosci 2001; 107:131–144Crossref, Medline, Google Scholar
34. Lee K-H, Williams LM, Haig A, Gordon E: Gamma (40 Hz) phase synchronicity and symptom dimensions in schizophrenia. Cognitive Neuropsychiatry 2003; 8:57–71Crossref, Google Scholar
35. Bell RC, Dudgeon P, McGorry PD, Jackson HJ: The dimensionality of schizophrenia concepts in first-episode psychosis. Acta Psychiatr Scand 1998; 97:334–342Crossref, Medline, Google Scholar
36. McGorry PD, Bell RC, Dudgeon PL, Jackson HJ: The dimensional structure of first episode psychosis: an exploratory factor analysis. Psychol Med 1998; 28:935–947Crossref, Medline, Google Scholar
37. The WAIS-III and WMS-III Technical Manual. San Antonio, Tex, Psychological Corp, 1997Google Scholar
38. Kaufman AS, Kaufman NL: K-BIT: Kaufman Brief Intelligence Test. Circle Pines, Minn, American Guidance Service, 1990Google Scholar
39. Nelson HE, O’Connell A: Dementia: the estimation of premorbid intelligence levels using the New Adult Reading Test. Cortex 1978; 14:234–244Crossref, Medline, Google Scholar
40. Robins LN, Wing J, Wittchen HU, Helzer JE, Babor TF, Burke J, Farmer A, Jablenski A, Pickens R, Regier DA, Sartorius N, Towle LH: The Composite International Diagnostic Interview: an epidemiologic instrument suitable for use in conjunction with different diagnostic systems and in different cultures. Arch Gen Psychiatry 1988; 45:1069–1077Crossref, Medline, Google Scholar
41. Williams LM, Gordon E, Wright J, Bahramali H: Late component ERPs are associated with three syndromes in schizophrenia. Int J Neurosci 2000; 105:37–52Crossref, Medline, Google Scholar
42. van Kammen DP, Marder SR: Dopamine receptor antagonists, in Comprehensive Textbook of Psychiatry, 6th ed, vol 2. Edited by Kaplan HI, Sadock BJ. Baltimore, Williams & Wilkins, 1995, pp 1987–2022Google Scholar
43. Lambert T: Lundbeck Utility for Neuroleptic Conversion. Melbourne, Australia, University of Melbourne, Multimedia in Psychiatry Unit, 1998Google Scholar
44. Kay SR, Opler LA, Fiszbein A: Positive and Negative Syndrome Scale (PANSS). North Tonawanda, NY, Multi-Health Systems, 1986Google Scholar
45. Blom JL, Anneveldt M: An electrode cap tested. Electroencephalogr Clin Neurophysiol 1982; 54:591–594Crossref, Medline, Google Scholar
46. Gratton G, Coles MG, Donchin E: A new method for off-line removal of ocular artifact. Electroencephalogr Clin Neurophysiol 1983; 55:468–484Crossref, Medline, Google Scholar
47. Haig AR, De Pascalis V, Gordon E: Peak gamma latency correlated with reaction time in a conventional oddball paradigm. Clin Neurophysiol 1999; 110:158–165Crossref, Medline, Google Scholar
48. Haig AR, Gordon E: Prestimulus EEG alpha phase synchronicity influences N100 amplitude and reaction time. Psychophysiology 1998; 35:591–595Crossref, Medline, Google Scholar
49. Slewa-Younan S, Gordon E, Harris AW, Haig AR, Brown KJ, Flor-Henry P, Williams LM: Sex differences in functional connectivity in first-episode and chronic schizophrenia patients. Am J Psychiatry 2004; 161:1595–1602Link, Google Scholar
50. Levin S: Frontal lobe dysfunctions in schizophrenia, I: eye movement impairments. J Psychiatr Res 1984; 18:27–55Crossref, Medline, Google Scholar
51. Traub RD, Whittington MA, Stanford IM, Jefferys JG: A mechanism for generation of long-range synchronous fast oscillations in the cortex. Nature 1996; 383:621–624Crossref, Medline, Google Scholar
52. Whittington MA, Traub RD, Jefferys JG: Synchronized oscillations in interneuron networks driven by metabotropic glutamate receptor activation. Nature 1995; 373:612–615Crossref, Medline, Google Scholar
53. Pierri JN, Chaudry AS, Woo T-UW, Lewis DA: Alterations in chandelier neuron axon terminals in the prefrontal cortex of schizophrenic subjects. Am J Psychiatry 1999; 156:1709–1719Abstract, Google Scholar
54. Busatto GF, Pilowsky LS, Costa DC, Ell PJ, David AS, Lucey JV, Kerwin RW: Correlation between reduced in vivo benzodiazepine receptor binding and severity of psychotic symptoms in schizophrenia. Am J Psychiatry 1997; 154:56–63; correction, 154:722Link, Google Scholar
55. Clementz BA, Blumenfeld LD, Cobb S: The gamma band response may account for poor P50 suppression in schizophrenia. Neuroreport 1997; 8:3889–3893Crossref, Medline, Google Scholar
56. Wright JJ, Kydd RR: Schizophrenia as a disorder of cerebral state transition. Aust NZ J Psychiatry 1986; 20:167–178Crossref, Medline, Google Scholar
57. Gordon E: Integrative psychophysiology. Int J Psychophysiol 2001; 42:95–108Crossref, Medline, Google Scholar
58. van Os J, Hanssen M, Bijl RV, Ravelli A: Strauss (1969) revisited: a psychosis continuum in the general population? Schizophr Res 2000; 45:11–20Crossref, Medline, Google Scholar
59. Peled A: Multiple constraint organization in the brain: a theory for schizophrenia. Brain Res Bull 1999; 49:245–250Crossref, Medline, Google Scholar
60. Bressler SL, Coppola R, Nakamura R: Episodic multiregional cortical coherence at multiple frequencies during visual task performance. Nature 1993; 366:153–156Crossref, Medline, Google Scholar
61. Gift TE, Strauss JS, Ritzler BA, Kokes RF, Harder DW: How diagnostic concepts of schizophrenia differ. J Nerv Ment Dis 1980; 168:3–8Crossref, Medline, Google Scholar
62. Johnstone EC, Crow TJ, Frith CD, Owens DG: The Northwick Park “functional” psychosis study: diagnosis and treatment response. Lancet 1988; 2:119–125Crossref, Medline, Google Scholar