Reduced Spinophilin But Not Microtubule-Associated Protein 2 Expression in the Hippocampal Formation in Schizophrenia and Mood Disorders: Molecular Evidence for a Pathology of Dendritic Spines
Abstract
OBJECTIVE: Aberrant synaptic connectivity may underlie the involvement of the hippocampus in schizophrenia. There is reasonable neuropathological evidence for a presynaptic pathology but few studies of the postsynaptic component. This study tested the hypothesis that hippocampal dendritic pathology is also present in schizophrenia. METHOD: Using in situ hybridization in sections of hippocampal formation from 10 patients with schizophrenia, 10 patients with mood disorders (three with bipolar disorder and seven with major depression), and 10 healthy comparison subjects, the authors examined the expression of two important dendritic genes: spinophilin, which serves as a marker of dendritic spines, and microtubule-associated protein 2 (MAP2), an overall dendritic marker. RESULTS: The patients with schizophrenia had lower levels of spinophilin mRNA in CA4 (hilus), CA3, the subiculum, and the entorhinal cortex than did the normal comparison subjects. The mood disorder group showed similar differences from the comparison group. MAP2 and cyclophilin mRNA did not differ between the groups in any subfield. CONCLUSIONS: Decreased spinophilin but unchanged MAP2 expression provides molecular evidence for a hippocampal dendritic pathology in schizophrenia that preferentially affects the spines. As spines are the target of most glutamatergic synapses, the data extend the evidence that excitatory synapses are particularly affected. Similar dendritic spine pathology may also occur in mood disorders.
Synaptic pathology has received increasing interest as a key feature of schizophrenia’s neuropathology (1–3) and possibly its pathogenesis (4–10) and genetic etiology (11). Most investigations of synaptic pathology in schizophrenia have used presynaptic proteins and their mRNAs as molecular markers, respectively providing information about the synaptic terminals and the neurons that give rise to them (12). Particularly in the hippocampal formation, there is relatively consistent evidence for low expression of genes for presynaptic proteins, such as synaptophysin (13–17). The precise anatomical distribution and neurochemical phenotype of the affected synapses remain largely unknown, although glutamatergic synapses may be preferentially involved (18–20), and the CA1 subfield may be spared more than the rest of Ammon’s horn and the subiculum (13, 15, 16).
The preceding data suggest that aberrant synaptic connectivity may contribute to hippocampal dysfunction in schizophrenia (21, 22). However, such inferences are premature without information about the status of the postsynaptic elements of the synapses. Hence, evaluation of hippocampal dendrites and dendritic spines (the protuberances on which most excitatory synapses are apposed) is necessary. To date, the only data of which we are aware are reports of decreased dendritic arborization and spine density in subicular pyramidal neurons (23) and reduced immunoreactivity of microtubule-associated protein 2 (MAP2), a dendritic protein, in some (24, 25) but not all (26, 27) studies. Thus, we examined the expression of genes for two dendritic proteins: MAP2 and spinophilin (neurabin II), a marker of the dendritic spine (28, 29). We used in situ hybridization, to allow the neurons expressing these genes to be studied and to be able to relate the subfield distribution of abnormalities to the reported presynaptic protein findings. We included a group of subjects with mood disorders, for which there is preliminary evidence of presynaptic (16, 30, 31) and dendritic (23) pathology.
Method
Subjects Studied
The cohort comprised 10 subjects with schizophrenia, 10 mood disorder patients (three with bipolar disorder and seven with major depression), and 10 normal comparison subjects. Characteristics are presented in Table 1, and the subjects have been described in detail previously (16). All brain tissue used in this study was obtained with informed consent from the legal next of kin. Briefly, diagnoses were determined through independent reviews of clinical records by two psychiatrists using DSM-IV criteria. Of the 10 patients with mood disorders, three had had bipolar disorder and had received neuroleptics; the other seven had had major depression and were neuroleptic naive. Their antidepressant and mood stabilizer histories were not available. All of the subjects with mood disorders died by suicide. All subjects had negative results on a toxicological screen for recent use of illicit drugs. Routine neuropathological examination excluded gross and microscopic evidence of neurodegenerative changes. From each subject a block of hippocampal formation was collected and frozen as described previously (32). Coronal cryostat sections were cut at 14 m, thaw-mounted on gelatin-coated slides, and stored at –80°C.
Riboprobes and Oligonucleotides
Spinophilin riboprobe templates tagged with T7/T3 promoter were generated by reverse transcriptase polymerase chain reaction (PCR). To avoid nonspecific amplification, a longer spinophilin cDNA was amplified first and used as a template for a second PCR amplification (nested PCR). From the human brain cDNA, a single spinophilin/neurabin II product with an expected size of 608 base pairs (bp) was amplified by using outer primer 5′CCTGGAGAATGGCAGCAC3′ (bp 978–994, forward) and outer primer 5′CGGTCTTGACGAAGATACCC3′ (bp 1566–1585, reverse). In the next round of amplification, we used the first cDNA fragment as a template and T7/T3-promoter-tagged primers 5′CAGAGATGCATAATACGACTCACTATAGGGAGAGGTAGATGAATCCAAGAAGGA3′ (sense, artificial T7 promoter) and 5′CCAAGCCTTCATTAACCCTCACTAAAGGGAGACAGGGAACAGCTCCAACCT3′ (antisense, artificial T3 promoter). A single band with an expected size of 421 bp (including promoters) was amplified and sequenced (identical to GenBank number AJ401189, bp 1128–1483). Antisense and sense riboprobes were generated by using either T7 or T3 polymerase, [35S]dUTP, and an in vitro transcription kit. The 35S-labeled riboprobes were labeled to a specific activity of 1.4×109 counts/minute per microgram and purified by ethanol precipitation.
For detection of MAP2 mRNA, an oligodeoxynucleotide probe was designed to be specific for bases 251–292 of human cDNA (33; GenBank accession number HSU89330) and targeted the 5′ region, which is conserved between transcript variants. Cyclophilin mRNA was also measured, as a housekeeping gene control, as described previously (34).
In Situ Hybridization Histochemistry
Six adjacent human brain sections were pretreated for in situ hybridization (35). In situ hybridization for MAP2 was performed as described previously (36). The sections were incubated overnight at 33°C, and postincubation washes were carried out at 55°C. The experimental controls for MAP2 in situ hybridization comprised the following: concurrent hybridization with sense strand probes, hybridization in the presence of 50-fold excess unlabeled probe, and pretreatment of sections with ribonuclease (20 μg/ml of RNase A at 37°C for 20 minutes). Riboprobe in situ hybridization for spinophilin and cyclophilin was conducted as previously described (34), with sense strand hybridization as the experimental control. After in situ hybridization, the sections were exposed to autoradiographic film (Biomax, Kodak, Rochester, N.Y.) for 18 days (MAP2), 5 days (spinophilin), or 40 hours (cyclophilin) along with carbon-14 microscales. Subsequently, the sections were coated in photographic emulsion, stored for 4 weeks at 4°C, developed, and lightly counterstained with cresyl violet.
Image Analysis
The abundance of each mRNA was measured, by an investigator blind to subject group, from densitometric analysis of autoradiographs by using Kontron KS400 software (Imaging Associates, Bicester, U.K.). The cytoarchitectonic boundaries of the hippocampal formation were delineated according to the method of Duvernoy (37). Measurements were taken over the dentate gyrus (granule cell and molecular layers), CA4 (hilus), the pyramidal cell layer of CA3, CA2, and CA1, the subiculum, and the gray matter of the entorhinal cortex. Twenty randomly selected areas were sampled over each hippocampal subfield from two sections per probe per subject. The mean value of the duplicate sections was used for statistical analysis. Optical densities were converted to radioactivity values (nanocuries per gram) by using carbon-14 microscales.
Statistical Analyses
Comparisons of diagnostic groups were made by using one-way analysis of variance for each subfield. Significant (p<0.05) group differences were followed up with post hoc least significant difference tests. Because of the small number of subjects with bipolar disorder, they were combined with those having major depression to form a single mood disorder group for the statistical analyses. Correlations of the mRNA levels with values for demographic variables, including age, tissue pH, and postmortem interval, were performed for all subjects with Spearman’s correlations. Correlations of mRNA levels with lifetime neuroleptic exposure and final neuroleptic dose (Table 1) were investigated in the schizophrenia cohort. Finally, correlations between the presynaptic marker synaptophysin (measured previously in this cohort; see reference 16) and spinophilin mRNA were investigated in all groups.
Results
Distributions of Spinophilin and MAP2 mRNA
The distributions of MAP2, spinophilin, and cyclophilin mRNAs in the human hippocampal formation are shown in parts A, B, and C, respectively, of Figure 1. The three transcripts were detectable in all subfields examined, in all subjects, with prominent expression over the granule cell layer of the dentate gyrus and the pyramidal neuron layer of the hippocampus.
Unlike most mRNAs, which remain in the cell body, MAP2 mRNA in the rat hippocampus is transported into proximal dendrites (38, 39). This also appears to occur in the human hippocampus, giving a fuzzy appearance in the inner molecular layer of the dentate gyrus and the stratum lucidum/radiatum of Ammon’s horn (part D in Figure 1), contrasting with the sharp edges of the stratum granulosum and stratum pyramidale seen for nondendritically transported mRNAs, such as cyclophilin mRNA (part F in Figure 1). The signal for spinophilin mRNA (part E in Figure 1) is also suggestive of dendritic localization; indeed, as in the rat (36), it is more extensive than that for MAP2 mRNA, with labeling throughout the molecular layer and over the strata lucidum, radiatum, lacunosum, and moleculare of Ammon’s horn. None of the experimental controls produced any detectable signal (part G in Figure 1 and data not shown). Inspection of the dipped sections confirmed the preceding assessments of mRNA distribution (data not shown).
Spinophilin mRNA in Schizophrenia and Mood Disorders
There was a significant effect of diagnosis on spinophilin mRNA abundance in CA4 (F=5.88, df=2, 29, p=0.008), CA3 (F=5.24, df=2, 29, p=0.01), the subiculum (F=3.66, df=2, 30, p=0.04), and the entorhinal cortex (F=5.13, df=2, 30, p=0.01). Compared to the healthy subjects, spinophilin mRNA was significantly lower in the patients with schizophrenia (CA4, –45%; CA3, –44%; subiculum, –24%; and entorhinal cortex, –27%) and in those with mood disorders (CA4, –39%; CA3, –32%; subiculum, –25%; and entorhinal cortex, –36%) (Figure 2). The schizophrenia and mood disorder groups did not differ from each other. Within the mood disorder group, the percentage differences from the healthy comparison subjects were similar in the group with bipolar disorder and the group with major depression (for CA4: bipolar, –43%; depressed, –37%; for CA3: bipolar, –37%; depressed, –30%; for the subiculum: bipolar, –23%; depressed, –26%; for the entorhinal cortex: bipolar, –45%; depressed, –32%).
Significant correlations between spinophilin mRNA and pH were observed in all subfields (r>0.49, N=30, p≤0.03) except CA1 (r=0.17, N=30, p=0.4). Postmortem interval correlated negatively with spinophilin mRNA in the granule cell layer (r=–0.38, N=30, p=0.03) and CA4 (r=–0.36, N=30, p=0.05). There were no correlations between spinophilin mRNA and lifetime neuroleptic exposure in the schizophrenia cohort (0.04<r<0.45, N=10, all p>0.2) or last recorded daily neuroleptic dose (range, –0.49<r<–0.03, N=10, all p>0.2).
Significant positive correlations between synaptophysin mRNA (data from reference 16) and spinophilin mRNA were observed in the whole group (r=0.65–0.75, p=0.01 to p=0.001), in the schizophrenia cohort (r=0.50–0.94, p=0.02 to p=0.005), and in the mood disorder group (r=0.70–0.90, p=0.04 to p=0.002).
MAP2 and Cyclophilin mRNAs in Schizophrenia and Mood Disorders
There was no significant diagnostic effect on MAP2 mRNA (Figure 2) (F<2.01, p>0.2) or cyclophilin mRNA (Figure 3) (F<1.27, p>0.3) in any hippocampal subfield, and there was no correlation of mRNA levels with neuroleptic medication exposure, in the schizophrenia group, or with age, pH, or postmortem interval, in the total group.
Discussion
Hypotheses that aberrant synaptic development and plasticity are important in schizophrenia (4–6, 9) are increasingly being supported by convergent empirical data from molecular and neuropathological studies (1, 3, 8, 17). However, most of the existing data regarding the hippocampal formation pertain to the presynaptic side of the synapse, and little is known about the key postsynaptic elements: dendrites and their spines. This study provides molecular evidence that the latter are also affected in schizophrenia, with a lower level of the mRNA encoding the dendritic spine protein spinophilin. This deficit was molecularly specific, in that no abnormality occurred in transcripts encoding the dendritic marker MAP2 and the housekeeping gene cyclophilin.
The simplest interpretation of the spinophilin reduction is that it reflects a loss of dendritic spines. This morphological interpretation is consistent with the findings of Rosoklija et al. (23) in the subiculum and suggests that similar changes occur in several other hippocampal subfields. It is also in keeping with experimental studies showing that alterations in dendritic spine number are accompanied by parallel changes in spinophilin expression (28, 40). A similar relationship pertains between MAP2 expression and overall dendritic extent (41–43); hence, our negative results for MAP2 suggest that the total dendritic tree of hippocampal neurons is not markedly altered in schizophrenia. An alternative interpretation of the reduced spinophilin gene expression in schizophrenia is that it reflects a decreased activity or plasticity of dendritic spines, rather than (or as well as) morphological change per se (7). This interpretation is plausible since spines are dynamic structures central to synaptic plasticity and affected by synaptic activity (44, 45) and spinophilin is implicated in these processes (26, 46, 47).
The deficits in hippocampal expression of genes for presynaptic proteins in this (16) and other (13, 15, 17, 30) groups of schizophrenia patients and the positive correlations between synaptophysin mRNA and spinophilin mRNA, in our total study group and in the disease groups separately, raise the question of how these facets of synaptic pathology are related. Regarding the direction of causality, precedents suggest that dendritic pathology is likely to be secondary to abnormal afferent innervation, rather than the other way around (48–51). However, consideration of the anatomical correspondence between pre- and postsynaptic abnormalities suggests that the relationship may be indirect: the synaptophysin mRNA studies (13, 15, 16) all show substantially lower levels in CA3, which provides the primary input to CA1 neurons via the Schaffer collaterals, but no difference in CA1. Hence, if there were a direct relationship between pre- and postsynaptic changes, spinophilin mRNA would likely be decreased in CA1 to accompany the loss of synaptophysin mRNA in CA3. In fact, spinophilin mRNA showed a subfield profile of abnormalities similar to that of synaptophysin mRNA, with CA1 again unaffected. Thus, an alternative explanation is that there are intrinsic differences in the vulnerability of CA3 and CA1 neurons to synaptic pathology, with those in CA1 being resistant to both presynaptic and dendritic involvement. One determinant of this differential vulnerability may be genetic, in that three of the putative schizophrenia susceptibility genes, neuregulin, dysbindin, and calcineurin gamma, show much lower expression in CA1 than in the rest of the hippocampal formation (unpublished observations). It may also be that, in schizophrenia, other CA1 afferents (e.g., commissural or monoaminergic) are unusually high and compensate for the low input from CA3 or that CA3 neurons are intrinsically more sensitive to fluctuations in gene expression.
Most glutamatergic (excitatory) synapses terminate on dendritic spines, and most axospinous synapses are glutamatergic. Hence, the present results support the view that, at least within the hippocampal formation, glutamatergic neurotransmission is particularly affected in schizophrenia (19, 52, 53). An interesting development is the recent report that the abundance of GluR2, a key non-N-methyl-d-aspartate receptor subunit, regulates dendritic spine formation in hippocampal neurons (54). Since GluR2 expression is reduced in hippocampal neurons of schizophrenia patients (55, 56), GluR2 may be part of the molecular cascade that leads to the dendritic spine pathology of schizophrenia. This correspondence of findings also emphasizes that, at the molecular level, there is a continuum, not a distinction, between structural and functional (neurochemical) aspects of neuropathology.
Reductions in spinophilin mRNA were also observed in the mood disorder group, consistent with initial morphological evidence for reduced dendritic spine density (23). This suggests considerable overlap between schizophrenia and mood disorders regarding involvement of dendritic spines. Whether this similarity of pathology reflects similar pathogenic processes, such as those already outlined, remains to be determined.
Several potential limitations of this study should be noted. First, the small number of subjects with bipolar disorder required us to group them with the subjects who had major depression in a single mood disorder group for the analyses. The possibility of different abnormalities of dendritic gene expression among mood disorder subtypes thus requires further study; however, inspection of our data did not provide any indication of this.
Second, autoradiographic film analyses can be confounded by differences in the packing density of neurons between the groups being compared. Although there is no good evidence that this occurs in the hippocampus in schizophrenia (1) or mood disorders (31), we investigated the possibility directly by doing a “per cell” analysis of spinophilin mRNA in CA4 and CA1 neurons, using the emulsion-dipped sections. This showed that spinophilin mRNA is lower in CA4 neurons but not in CA1 neurons in schizophrenia (data not shown), confirming the film results.
Third, medication might have contributed to, or masked, differences between diagnostic groups. However, there were no correlations of the mRNAs with either lifetime or recent neuroleptic exposure, and chronic haloperidol and chlorpromazine treatment have no effect on spinophilin mRNA in the rat hippocampus (36). It also seems unlikely that occult substance misuse among the patients confounded the results, since the patients had negative toxicology results and since psychostimulants increase, not decrease, dendritic spines (57). A similar argument pertains to the possible effect of antidepressants and mood stabilizers, which increase spine densities in antidepressant-treated animals (31, 58).
Fourth, we found correlations of spinophilin mRNA with brain pH and, less clearly, with postmortem interval. However, these variables did not differ significantly between groups, and all the positive results remained significant when pH and postmortem interval were included as covariates in an analysis of covariance (data not shown).
Finally, a different limitation comes from the fact that we measured only the mRNAs and not the proteins. This decision was made because of the existence of multiple isoforms and phosphorylation states, which complicates quantitative antibody-based methods (26, 27), because the proteins may be sensitive to perimortem degradation (59), and also because of limited tissue availability. It is reassuring that prior studies have shown a good correspondence between protein and mRNA levels for several dendritic proteins (42, 60, 61). Nevertheless, evaluation of both types of gene product will be desirable in future work, which is also needed to confirm our observations and extend them to other brain regions wherein dendritic pathology has been reported in schizophrenia (62–64).
In conclusion, our results provide molecular evidence for a postsynaptic component of glutamatergic synaptic pathology in the hippocampal formation in schizophrenia and mood disorders. Decreased spinophilin expression suggests that the structural and functional integrity of dendritic spines is compromised. The combination of presynaptic and postsynaptic abnormalities may represent an important component of these disorders.
![]() |
Presented in part at the Ninth International Congress on Schizophrenia Research, Colorado Springs, Colo., March 29 to April 2, 2003, and at the 2003 summer meeting of the British Association of Psychopharmacology, Cambridge, U.K., July 21–23, 2003. Received Sept. 23, 2003; revision received Dec. 17, 2003; accepted Jan. 9, 2004. From the Department of Psychiatry, University of Oxford and Warneford Hospital; and the Clinical Brain Disorders Branch, Intramural Research Program, NIMH, Bethesda, Md. Address reprint requests to Dr. Law, University Department of Psychiatry, Neurosciences Building, Warneford Hospital, Oxford OX3 7JX, U.K.; [email protected] (e-mail). Supported by a Stanley Medical Research Institute Centre award to Dr. Harrison, a Wellcome Trust Biomedical Collaboration grant to Drs. Kleinman and Harrison, and a National Alliance for Research on Schizophrenia and Depression Young Investigator Award to Dr. Weickert. The authors thank Benjamin McClintock for technical assistance.
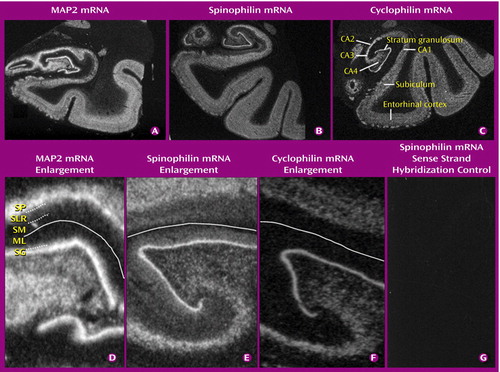
Figure 1. Autoradiographic Images Showing the Distributions of Microtubule-Associated Protein 2 (MAP2), Spinophilin, and Cyclophilin mRNAs in the Human Hippocampal Formationa
aPart C identifies the subfields of the hippocampal formation. Parts D–F are enlarged images of the dentate gyrus and adjacent hippocampus and illustrate dendritic localization of MAP2 and spinophilin mRNAs (see text). Part D: SP, stratum pyramidale; SLR, stratum lucidum/radiatum; SM, stratum moleculare; ML, molecular layer; SG, stratum granulosum (granule cell layer). Part D shows MAP2 mRNA labeling in the inner part of the molecular layer of the dentate gyrus (dotted line) and in the stratum lucidum/radiatum of Ammon’s horn (dotted line), below the stratum pyramidale. The solid line shows the position of the hippocampal fissure. Part E shows spinophilin mRNA labeling throughout the molecular layer and stratum lucidum/radiatum and extending into the stratum moleculare of Ammon’s horn. Part F shows no cyclophilin mRNA labeling over the molecular layer, stratum lucidum/radiatum, or stratum moleculare.
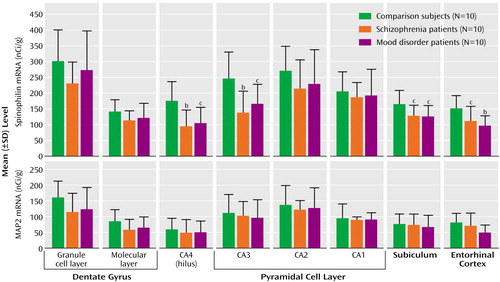
Figure 2. Spinophilin mRNA and Microtubule-Associated Protein 2 (MAP2) mRNA in the Hippocampal Formation of Healthy Comparison Subjects, Patients With Schizophrenia, and Patients With Mood Disordersa
aOf the patients with mood disorders, seven had major depression and three had bipolar disorder.
bSignificantly different from level of comparison subjects (p<0.01, least significant difference test).
cSignificantly different from level of comparison subjects (p<0.05, least significant difference test).
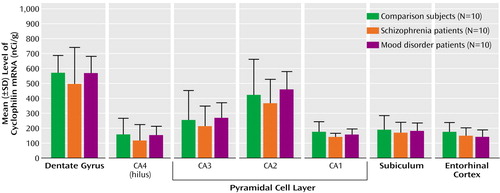
Figure 3. Cyclophilin mRNA in the Hippocampal Formation of Healthy Comparison Subjects, Patients With Schizophrenia, and Patients With Mood Disordersa
aOf the patients with mood disorders, seven had major depression and three had bipolar disorder.
1. Harrison PJ: The neuropathology of schizophrenia: a critical review of the data and their interpretation. Brain 1999; 122:593–624Crossref, Medline, Google Scholar
2. Selemon LD, Goldman-Rakic PS: The reduced neuropil hypothesis: a circuit based model of schizophrenia. Biol Psychiatry 1999; 45:17–25Crossref, Medline, Google Scholar
3. Honer WG, Young C, Falkai P: Synaptic pathology, in The Neuropathology of Schizophrenia: Progress and Interpretation. Edited by Harrison PJ, Roberts GW. Oxford, UK, Oxford University Press, 2000, pp 105–136Google Scholar
4. Feinberg I: Schizophrenia: caused by a fault in programmed synaptic elimination during adolescence? J Psychiatr Res 1982; 17:319–334Crossref, Medline, Google Scholar
5. Stevens JR: Abnormal reinnervation as a basis for schizophrenia: a hypothesis. Arch Gen Psychiatry 1992; 49:238–243Crossref, Medline, Google Scholar
6. McGlashan TH, Hoffman RE: Schizophrenia as a disorder of developmentally reduced synaptic connectivity. Arch Gen Psychiatry 2000; 57:637–648Crossref, Medline, Google Scholar
7. Costa E, Davis J, Grayson DR, Guidotti A, Pappas GD, Pesold C: Dendritic spine hypoplasticity and downregulation of reelin and GABAergic tone in schizophrenia vulnerability. Neurobiol Dis 2001; 8:723–742Crossref, Medline, Google Scholar
8. Mirnics K, Middleton FA, Lewis DA, Levitt P: Analysis of complex brain disorders with gene expression microarrays: schizophrenia as a disease of the synapse. Trends Neurosci 2001; 24:479–486Crossref, Medline, Google Scholar
9. Moises HW, Zoega T, Gottesman II: The glial growth factors deficiency and synaptic destabilization hypothesis of schizophrenia. BMC Psychiatry 2002; 2:8Crossref, Medline, Google Scholar
10. Frankle WG, Lerma J, Laruelle M: The synaptic hypothesis of schizophrenia. Neuron 2003; 39:205–216Crossref, Medline, Google Scholar
11. Harrison PJ, Owen MJ: Genes for schizophrenia? recent findings and their pathophysiological implications. Lancet 2003; 361:417–419Crossref, Medline, Google Scholar
12. Eastwood SL, Cairns NJ, Harrison PJ: Synaptophysin gene expression in schizophrenia: investigations of synaptic pathology in the cerebral cortex. Br J Psychiatry 2000; 176:236–242Crossref, Medline, Google Scholar
13. Eastwood SL, Burnet PWJ, Harrison PJ: Altered synaptophysin expression as a marker of synaptic pathology in schizophrenia. Neuroscience 1995; 66:309–319Crossref, Medline, Google Scholar
14. Eastwood SL, Harrison PJ: Decreased synaptophysin in the medial temporal lobe in schizophrenia demonstrated using immunoautoradiography. Neuroscience 1995; 69:339–343Crossref, Medline, Google Scholar
15. Eastwood SL, Harrison PJ: Detection and quantification of hippocampal synaptophysin messenger RNA in schizophrenia using autoclaved, formalin-fixed, paraffin wax-embedded sections. Neuroscience 1999; 93:99–106Crossref, Medline, Google Scholar
16. Webster MJ, Weickert CS, Herman MM, Hyde TM, Kleinman JE: Synaptophysin and GAP-43 mRNA levels in the hippocampus of subjects with schizophrenia. Schizophr Res 2001; 49:89–98Crossref, Medline, Google Scholar
17. Harrison PJ, Eastwood SL: Neuropathological studies of synaptic connectivity in the hippocampal formation in schizophrenia. Hippocampus 2001; 11:508–519Crossref, Medline, Google Scholar
18. Harrison PJ, Eastwood SL: Preferential involvement of excitatory neurons in medial temporal lobe in schizophrenia. Lancet 1998; 352:1669–1673Crossref, Medline, Google Scholar
19. Harrison PJ, Law AJ, Eastwood SL: Glutamate receptors and transporters in the hippocampus in schizophrenia. Ann NY Acad Sci USA 2003; 1003:94–101Crossref, Medline, Google Scholar
20. Sawada K, Barr AM, Takahashi S, Arima K, Falkai P, Phillips A, Honer WG: Complexins I and II in hippocampus in schizophrenia (abstract). Schizophr Res 2003; 60(suppl):74–75Google Scholar
21. Weinberger DR: Cell biology of the hippocampal formation in schizophrenia. Biol Psychiatry 1999; 45:395–402Crossref, Medline, Google Scholar
22. Heckers S: Neuroimaging studies of the hippocampus in schizophrenia. Hippocampus 2001; 11:520–528Crossref, Medline, Google Scholar
23. Rosoklija G, Toomayan G, Ellis SP, Keilp J, Mann JJ, Latov N, Hays AP, Dwork AJ: Structural abnormalities of subicular dendrites in subjects with schizophrenia and mood disorders: preliminary findings. Arch Gen Psychiatry 2000; 57:349–356Crossref, Medline, Google Scholar
24. Arnold SE, Lee VM, Gur RE, Trojanowski JQ: Abnormal expression of two microtubule-associated proteins (MAP2 and MAP5) in specific subfields of the hippocampal formation in schizophrenia. Proc Natl Acad Sci USA 1991; 88:10850–10854Crossref, Medline, Google Scholar
25. Rosoklija G, Mancevski B, Keilp J, Ilievsk B, Duma A, Filipovska A, Dwork AJ: Structural abnormalities of subicular dendrites in schizophrenia (abstract). Schizophr Res 2002; 53(suppl):108Google Scholar
26. Cotter D, Kerwin R, Doshi B, Martin CS, Everall IP: Alterations in hippocampal non-phosphorylated MAP2 protein expression in schizophrenia. Brain Res 1997; 765:238–246Crossref, Medline, Google Scholar
27. Cotter D, Wilson S, Roberts E, Kerwin R, Everall IP: Increased dendritic MAP2 expression in the hippocampus in schizophrenia. Schizophr Res 2000; 41:313–323Crossref, Medline, Google Scholar
28. Allen PB, Ouimet CC, Greengard P: Spinophilin, a novel protein phosphatase 1 binding protein localized to dendritic spines. Proc Natl Acad Sci USA 1997; 94:9956–9961Crossref, Medline, Google Scholar
29. Alves SE, Hoskin E, Lee SJ, Brake WG, Ferguson D, Luine V, Allen PB, Greengard P, McEwen BS: Serotonin mediates CA1 spine density but is not crucial for ovarian steroid regulation of synaptic plasticity in the adult rat dorsal hippocampus. Synapse 2002; 45:143–151Crossref, Medline, Google Scholar
30. Eastwood SL, Harrison PJ: Hippocampal synaptic pathology in schizophrenia, bipolar disorder and major depression: a study of complexin mRNAs. Mol Psychiatry 2000; 5:425–432Crossref, Medline, Google Scholar
31. Harrison PJ: The neuropathology of primary mood disorder. Brain 2002; 125:1428–1449Crossref, Medline, Google Scholar
32. Kleinman JE, Hyde TM, Herman MM: Methodological issues in the neuropathology of mental illness, in Psychopharmacology: The Fourth Generation of Progress. Edited by Bloom FE, Kupfer DJ. New York, Raven Press, 1995, pp 859–864Google Scholar
33. Albala JS, Kalcheva N, Shafit-Zagardo B: Characterization of the transcripts encoding two isoforms of human microtubule-associated protein-2 (MAP-2). Gene 1993; 136:377–378Crossref, Medline, Google Scholar
34. Webster MJ, Weickert CS, Herman MM, Kleinman JE: BDNF mRNA expression during postnatal development, maturation and aging of the human prefrontal cortex. Brain Res Dev Brain Res 2002; 139:139–150Crossref, Medline, Google Scholar
35. Law AJ, Deakin JF: Asymmetrical reductions of hippocampal NMDAR1 glutamate receptor mRNA in the psychoses. Neuroreport 2001; 12:2971–2974Crossref, Medline, Google Scholar
36. Law AJ, Hutchinson LJ, Burnet PWJ, Harrison PJ: Antipsychotics increase microtubule-associated protein 2 mRNA but not spinophilin mRNA in the rat hippocampus and cortex. J Neurosci Res 2004; 76:376–382Crossref, Medline, Google Scholar
37. Duvernoy HM: The Human Hippocampus: An Atlas of Applied Anatomy. Munich, Bergmann Verlag, 1988Google Scholar
38. Tucker RP, Garner CC, Matus A: In situ localization of microtubule-associated protein mRNA in the developing and adult rat brain. Neuron 1989; 2:1245–1256Crossref, Medline, Google Scholar
39. Steward O, Wallace CS: mRNA distribution within dendrites: relationship to afferent innervation. J Neurobiol 1995; 26:447–449Crossref, Medline, Google Scholar
40. Amateau SK, McCarthy MM: A novel mechanism of dendritic spine plasticity involving estradiol induction of prostaglandin-E2. J Neurosci 2002; 22:8586–8596Crossref, Medline, Google Scholar
41. Represa A, Pollard H, Moreau J, Ghilini G, Khrestchatisky M, Ben Ari Y: Mossy fiber sprouting in epileptic rats is associated with a transient increased expression of alpha-tubulin. Neurosci Lett 1993; 156:149–152Crossref, Medline, Google Scholar
42. Pollard H, Khrestchatisky M, Moreau J, Ben Ari Y, Represa A: Correlation between reactive sprouting and microtubule protein expression in epileptic hippocampus. Neuroscience 1994; 61:773–787Crossref, Medline, Google Scholar
43. Montgomery MM, Dean AF, Taffs F, Stott EJ, Lantos PL, Luthert PJ: Progressive dendritic pathology in cynomolgus macaques infected with simian immunodeficiency virus. Neuropathol Appl Neurobiol 1999; 25:11–19Crossref, Medline, Google Scholar
44. Hering H, Sheng M: Dendritic spines: structure, dynamics and regulation. Nat Rev Neurosci 2001; 2:880–888Crossref, Medline, Google Scholar
45. Kasai H, Matsuzaki M, Noguchi J, Yasumatsu N, Nakahara H: Structure-stability-function relationships of dendritic spines. Trends Neurosci 2003; 26:360–368Crossref, Medline, Google Scholar
46. Stephens DJ, Banting G: In vivo dynamics of the F-actin-binding protein neurabin-II. Biochem J 2000; 345(part 2):185–194Google Scholar
47. Feng J, Yan Z, Ferreira A, Tomizawa K, Liauw JA, Zhuo M, Allen PB, Ouimet CC, Greengard P: Spinophilin regulates the formation and function of dendritic spines. Proc Natl Acad Sci USA 2000; 97:9287–9292Crossref, Medline, Google Scholar
48. Fiala JC, Spacek J, Harris KM: Dendritic spine pathology: cause or consequence of neurological disorders? Brain Res Brain Res Rev 2002; 39:29–54Crossref, Medline, Google Scholar
49. Miwa C, Ueki A, Shinjo H, Simode H, Morita Y: Long-term synaptic alteration in the rat hippocampal CA3 field following an entorhinal cortex lesion. Psychiatry Clin Neurosci 2001; 55:573–578Crossref, Medline, Google Scholar
50. Selkoe DJ: Alzheimer’s disease is a synaptic failure. Science 2002; 298:789–791Crossref, Medline, Google Scholar
51. Wong RO, Ghosh A: Activity-dependent regulation of dendritic growth and patterning. Nat Rev Neurosci 2002; 3:803–812Crossref, Medline, Google Scholar
52. Tsai G, Passani LA, Slusher BS, Carter R, Baer L, Kleinman JE, Coyle JT: Abnormal excitatory neurotransmitter metabolism in schizophrenic brains. Arch Gen Psychiatry 1995; 52:829–836Crossref, Medline, Google Scholar
53. Tamminga C: Glutamatergic aspects of schizophrenia. Br J Psychiatry Suppl 1999; 37:12–15Medline, Google Scholar
54. Passafaro M, Nakagawa T, Sala C, Sheng M: Induction of dendritic spines by an extracellular domain of AMPA receptor subunit GluR2. Nature 2003; 424:677–681Crossref, Medline, Google Scholar
55. Eastwood SL, McDonald B, Burnet PW, Beckwith JP, Kerwin RW, Harrison PJ: Decreased expression of mRNAs encoding non-NMDA glutamate receptors GluR1 and GluR2 in medial temporal lobe neurons in schizophrenia. Brain Res Mol Brain Res 1995; 29:211–223Crossref, Medline, Google Scholar
56. Eastwood SL, Kerwin RW, Harrison PJ: Immunoautoradiographic evidence for a loss of alpha-amino-3-hydroxy-5-methyl-4-isoxazole propionate-preferring non-N-methyl-D-aspartate glutamate receptors within the medial temporal lobe in schizophrenia. Biol Psychiatry 1997; 41:636–643Crossref, Medline, Google Scholar
57. Robinson TE, Gorny G, Mitton E, Kolb B: Cocaine self-administration alters the morphology of dendrites and dendritic spines in the nucleus accumbens and neocortex. Synapse 2001; 39:257–266Crossref, Medline, Google Scholar
58. Norrholm SD, Ouimet CC: Altered dendritic spine density in animal models of depression and in response to antidepressant treatment. Synapse 2001; 42:151–163Crossref, Medline, Google Scholar
59. Schwab C, Bondada V, Sparks DL, Cahan LD, Geddes JW: Postmortem changes in the levels and localization of microtubule-associated proteins (tau, MAP2 and MAP1B) in the rat and human hippocampus. Hippocampus 1994; 4:210–225Crossref, Medline, Google Scholar
60. Eastwood SL, Heffernan J, Harrison PJ: Chronic haloperidol treatment differentially affects the expression of synaptic and neuronal plasticity-associated genes. Mol Psychiatry 1997; 2:322–329Crossref, Medline, Google Scholar
61. Pei Q, Burnet PJ, Zetterstrom TS: Changes in mRNA abundance of microtubule-associated proteins in the rat brain following electroconvulsive shock. Neuroreport 1998; 9:391–394Crossref, Medline, Google Scholar
62. Garey LJ, Ong WY, Patel TS, Kanani M, Davis A, Mortimer AM, Barnes TR, Hirsch SR: Reduced dendritic spine density on cerebral cortical pyramidal neurons in schizophrenia. J Neurol Neurosurg Psychiatry 1998; 65:446–453Crossref, Medline, Google Scholar
63. Glantz LA, Lewis DA: Decreased dendritic spine density on prefrontal cortical pyramidal neurons in schizophrenia. Arch Gen Psychiatry 2000; 57:65–73Crossref, Medline, Google Scholar
64. Broadbelt K, Byne W, Jones LB: Evidence for a decrease in basilar dendrites of pyramidal cells in schizophrenic medial prefrontal cortex. Schizophr Res 2002; 58:75–81Crossref, Medline, Google Scholar