Thalamic Dysfunction in Schizophrenia Suggested by Whole-Night Deficits in Slow and Fast Spindles
Abstract
Objective:
Slow waves and sleep spindles are the two main oscillations occurring during non-REM sleep. While slow oscillations are primarily generated and modulated by the cortex, sleep spindles are initiated by the thalamic reticular nucleus and regulated by thalamo-reticular and thalamo-cortical circuits. In a recent high-density EEG study, the authors found that 18 medicated schizophrenia patients had reduced sleep spindles, compared with healthy and depressed subjects, during the first non-REM episode. In the present study, the authors investigated whether spindle deficits were present in a larger sample of schizophrenia patients, were consistent across the night, were related to antipsychotic medications, and were suggestive of impairments in specific neuronal circuits.
Method:
Whole-night high-density EEG recordings were performed in 49 schizophrenia patients, 20 nonschizophrenia patients receiving antipsychotic medication, and 44 healthy subjects. In addition to sleep spindles, several parameters of slow waves were assessed.
Results:
Schizophrenia patients had whole-night deficits in spindle power (12–16 Hz) and in slow (12–14 Hz) and fast (14–16 Hz) spindle amplitude, duration, number, and integrated activity in the prefrontal, centroparietal, and temporal regions. Integrated spindle activity and spindle number had the largest effect sizes (effect size: ≥2.21). In contrast, no slow wave deficits were found in schizophrenia patients.
Conclusions:
These results indicate that spindle deficits can be reliably established in schizophrenia, are stable across the night, are unlikely to be due to antipsychotic medications, and point to deficits in the thalamic reticular nucleus and thalamo-reticular circuits.
Schizophrenia is a disabling, chronic illness, which has marked socioeconomic effects on the patients and the entire community (1). Despite considerable efforts, the neurobiology of schizophrenia remains elusive (2), and its diagnosis is based exclusively on descriptive symptoms. To date, a symptom-based approach has not increased our understanding of schizophrenia. This is because clinical symptoms vary across the course of the illness, thus complicating the identification of underlying neurobio-logical mechanisms (3).
An alternative approach employed in schizophrenia research involves measuring cognitive/neurophysiological parameters in healthy subjects and schizophrenia patients (4). Deficits in these parameters can reveal dysfunctions in neuronal mechanisms that are stable over time and largely symptom-independent in individuals with schizophrenia (5). Moreover, these dysfunctions likely implicate specific brain circuits, which may help to identify better genetic/molecular targets for the diagnosis and treatment of schizophrenia (6).
Sleep offers important advantages for investigating possible dysfunctions in brain circuits in schizophrenia patients. Sleep recordings minimize waking-related confounding factors, including fluctuation in attention, reduced cognitive ability, and presence of active symptoms. Additionally, the two main nonrapid eye movement (non-REM) sleep rhythms, slow waves and spindles, reflect the activity of complementary thalamo-cortical circuits. Slow waves are 1-Hz oscillations characterized by large amplitude, positive-negative defiections, which are generated by cortical neurons and propagated by intracortical and cortico-thalamo-cortical circuits (7). Sleep spindles are waxing/waning 12- to 16-Hz oscillations, which are initiated by the thalamic reticular nucleus and regulated by thalamo-thalamic and thalamo-cortical circuits (8). Thus, the identification of reduced slow wave and/or spindle parameters may help determine deficits in specific brain circuits in schizophrenia.
We previously found that sleep spindles were reduced in the first non-REM episode of 18 medicated schizophrenia patients (9). Schizophrenia patients showed deficits in spindle amplitude, duration, and number relative to healthy and depressed subjects. Furthermore, integrated spindle activity, which was calculated by integrating the amplitude of each spindle over time, was reduced in 16 of 18 schizophrenia patients (9).
In the present study, we extended these results by performing whole-night high-density EEG recordings in 49 schizophrenia patients. To assess medication effects on spindles, we investigated spindle activity in nonschizophrenia patients receiving antipsychotics. Additionally, to establish whether spindle deficits primarily reflect impairments of thalamic reticular nucleus and intrathalamic circuits, we analyzed several sleep slow wave parameters.
Method
Participants
Forty-nine schizophrenia patients, 44 healthy subjects, and 20 nonschizophrenia patients receiving antipsychotic medication were recruited. All subjects were between 18 and 55 years of age. Exclusion criteria were substance abuse/dependence within the last 6 months, identifiable neurological disorders, or diagnosed sleep disorders. Healthy subjects with first-degree relatives with psychiatric diagnoses were also excluded. Demographic and clinical variables were similar across groups except for gender, body mass index, and medication doses (see Table 1 in the data supplement accompanying the online version of this article). A psychiatrist interviewed all participants and administered the Structured Clinical Interview for DSM-IV to confirm or exclude psychiatric diagnoses and further evaluated schizophrenia patients with the Positive and Negative Syndrome Scale (PANSS). Schizophrenia patients were diagnosed as paranoid (N=35), undifferentiated (N=5), disorganized (N=5), or residual subtype (N=4). They were receiving second-generation (N=35), first- and second-generation (N=10), or first-generation (N=4) antipsychotics. They were also receiving benzodiazepines (N=10), antidepressants (N=22), and mood stabilizers (N=11). Patients with schizophrenia were outpatients with a mean duration of illness of 15 years (SD=8) and a mean total PANSS score of 88.5 (SD=12.7).
Variable | Healthy Comparison Subjects (N=44) | Schizophrenia Patients (N=49) | Medicated Comparison Subjects (N=20) | |||
---|---|---|---|---|---|---|
Mean | SD | Mean | SD | Mean | SD | |
Total sleep time (minutes) | 447.6 | 62.0 | 472.5 | 91.0 | 465.8 | 75.8 |
Sleep onset latency (minutes)b | 14.1 | 1.5 | 26.7 | 3.6 | 20.5 | 4.3 |
Sleep maintenance (%)c | 90.8 | 9.0 | 91.2 | 10.5 | 92.8 | 9.6 |
Non-REM stage 1 (%) | 6.3 | 1.3 | 5.7 | 1.3 | 7.9 | 1.9 |
Non-REM stage 2 (%) | 51.6 | 4.4 | 55.5 | 5.6 | 52.5 | 6.3 |
Non-REM stage 3 (%) | 21.5 | 3.6 | 19.9 | 5.3 | 19.6 | 5.0 |
REM (%) | 20.6 | 2.5 | 18.9 | 2.65 | 20.0 | 2.8 |
TABLE 1. Sleep Architecture in Schizophrenia Patients, Nonschizophrenia Patients Receiving Antipsychotic Medication, and Healthy Subjectsa
Nonschizophrenia patients had various psychiatric diagnoses as follows: major depression (N=4), bipolar affective disorder (N=10), posttraumatic stress disorder (N=2), panic disorder (N=2), and generalized anxiety disorder (N=2). Seventeen were receiving second-generation antipsychotics, and three were receiving first-generation antipsychotics. Additionally, five were receiving benzodiazepines, seven were receiving antidepressants, and three were receiving mood stabilizers.
EEG Recordings
Whole-night high-density EEGs were performed with 256-electrode nets designed to improve electrode contact with the scalp, thereby enabling long-duration recordings (EGI, Eugene, Oregon). EEGs were performed at the Wisconsin Psychiatric Institute and Clinic. The study was approved by the University of Wisconsin's Institutional Review Board. After a complete description of the study, written informed consent was obtained. The participants were then placed in a sleep suite and allowed to sleep at their self-reported bedtime until morning.
EEG Analysis
EEG signals were band-passed (0.5–50 Hz) and digitized at 500 Hz. After excluding electrodes located on the neck/face region and channels with impedances >150 KOhms, 175–185 channels per subject were retained. Sleep stages were scored on C3A2 and C4A1 derivations by two authors, who were blind to subjects' identity, using the American Academy of Sleep Medicine criteria (10). Interrater reliability was >90%. Artifacts were visually excluded during scoring. Additional artifacts were removed, rejecting 20-second epochs, which exceeded thresholds based on the mean power for each channel in 0.75- to 4.5-Hz bands and 20-to 30-Hz bands. Signals were then re-referenced to the average for all included channels. This montage was used for computing power spectra of non-REM epochs (9).
Spindle Analysis
Non-REM epochs were filtered between 11 and 16 Hz, and rectified filtered signals were used as time series for each channel. Because signal amplitude varied considerably across channels, thresholds relative to the mean amplitude of each channel were used. For each spindle, the amplitude was the maximum above an upper threshold, while the beginning and end were the points preceding or following this maximum (≥0.25 second) when the amplitude dropped below a lower threshold. The lower threshold was set at two times the mean amplitude of the channel signal. The upper threshold was set at the lesser of either eight times the mean amplitude or the average between this value and an average upper threshold value calculated for each channel in a group of healthy subjects (N=12). For each spindle, the duration, amplitude, number, and integrated spindle activity were measured (9). To characterize spindles in slow (12–14 Hz) and fast (14–16 Hz) ranges, spindle parameters were calculated from non-REM data filtered in these two frequency bands. Slow and fast spindles have different topography and likely involve different thalamo-cortical modules. By exploring both ranges, we investigated whether spindle deficits were generalized or rather restricted to specific thalamo-cortical modules.
Slow Wave Analysis
To detect slow waves, we employed a recently developed procedure (11). Briefly, each EEG signal was referenced to the average of the two earlobes and band-pass filtered at 0.5–4.0 Hz. Then, waves were detected as negative defiections between two zero crossings. The zero crossing detection was chosen because of the high degree of variability in positive defiections relative to the stability of negative defiections. Only waves with 0.25- to 1.0-second consecutive zero crossings detected in artifact-free non-REM epochs were considered slow waves. Total incidence of waves, negative peak amplitude, and average slopes for each wave were determined. Average slopes were defined as the negative peak amplitude divided by the time from the previous zero crossing (down slopes) or until the next zero crossing (up slopes).
Statistical Analyses
To compare demographic characteristics, sleep architecture, and EEG power between groups, one-way analyses of variance (ANOVAs) followed by post hoc unpaired t tests were performed. Group differences in spindle amplitude, duration, number, and integrated activity or in slow wave incidence, amplitude, and down and up slopes were assessed with statistical nonparametric mapping (12). For spindle parameters, we also calculated Cohen's d (13). Finally, we performed correlation analyses between spindles and several clinical parameters of schizophrenia patients, including PANSS scores.
Results
Sleep Architecture
The three groups did not differ in sleep architecture (Table 1), except for sleep latency (one-way ANOVA: F=4.8, df=2, 110, p=0.01). Specifically, post hoc t tests showed increased sleep latency in schizophrenia patients relative to healthy comparison subjects (p<0.01). No difference was found between schizophrenia patients and nonschizophrenia patients receiving antipsychotics or between patients and healthy subjects (p≥0.1).
EEG Power Analysis
Whole-night non-REM EEG power was calculated between 0.5 and 40 Hz for the three groups (Figure 1). One-way ANOVA revealed group differences in the spindle range (12–16 Hz; F≥3.5, df=2,110, p≤0.03). Post hoc t tests showed power reductions in schizophrenia patients compared with healthy subjects (12–16 Hz, p≤0.03) and non-schizophrenia patients receiving antipsychotics (12–16 Hz, p≤0.02) but no difference between nonschizophrenia patients and healthy subjects. Power deficits were present in each sleep cycle and restricted to spindle range.
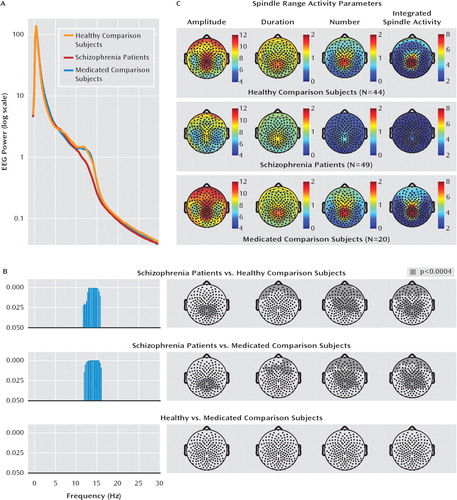
FIGURE 1. Spindle Range Activity Parameters in Schizophrenia Patients, Nonschizophrenia Patients Receiving Antipsychotic Medication, and Healthy Subjectsa
a Schizophrenia patients had deficits in spindle range (12–16 Hz) power and other spindle parameters. A) Mean EEG power spectra in 0.25-Hz resolution of whole-night non-REM sleep among schizophrenia patients and medicated (nonschizophrenia patients receiving antipsychotics) and healthy comparison subjects is shown. One-way ANOVA revealed significant differences between the three groups, from 12 to 16 Hz (F>3.61, df=2, 106, p<0.05). B) Post hoc t tests showed statistically significant differences between schizophrenia patients and healthy comparison subjects from 12.5 to 15.75 Hz and between schizophrenia patients and medicated comparison subjects from 12 to 16 Hz. (blue bars). C) Color plots depict the topography of several spindle parameters in schizophrenia patients, healthy comparison subjects, and medicated comparison subjects. Noncolor plots depict topographic distribution of the electrodes showing a significant reduction (gray area, statistical nonparametric mapping [p<0.0004]) in spindle parameters in schizophrenia patients versus healthy comparison subjects, schizophrenia patients versus medicated comparison subjects, and healthy versus medicated comparison subjects.
Spindle Analysis
An initial analysis was performed for spindle amplitude, duration, number, and integrated activity in 12–16 Hz; the frequency range decreased in schizophrenia patients. The topography of each parameter was similar across groups, with peaks in prefrontal and centroparietal areas. Spindle parameters in these regions were markedly reduced in schizophrenia patients (Figure 1). Spindle amplitude was decreased in schizophrenia patients relative to healthy comparison subjects and medicated nonschizophrenia comparison subjects in centroparietal areas (statistical nonparametric mapping, p<0.0004), while spindle duration was decreased in schizophrenia patients relative to healthy and medicated comparison subjects in prefrontal regions (statistical nonparametric mapping, p<0.0002). Schizophrenia patients also showed deficits in spindle number and integrated activity relative to healthy (statistical nonparametric mapping, p<0.0001) and medicated comparison subjects (statistical nonpara-metric mapping, p<0.0001) in prefrontal and centroparietal regions. No difference was found between healthy and medicated comparison subjects for any spindle parameter.
Additional analyses were performed for slow (12–14 Hz) and fast (14–16 Hz) spindles. Schizophrenia patients showed deficits in slow spindle duration, number, and integrated activity in prefrontal regions relative to both healthy and medicated comparison subjects (statistical nonparametric mapping, p≤0.001 [Figure 2]). We found no difference in slow spindle amplitude across the three groups or between healthy and medicated comparison subjects in other slow spindle parameters. Deficits in fast spindle duration (prefrontal), amplitude (centroparietal), and number and integrated activity (both prefrontal and centroparietal) were found in the schizophrenia group relative to the healthy and medicated comparison groups (statistical nonparametric mapping, p≤0.001). Additionally, schizophrenia patients had reduced fast spindle number and integrated activity in the left temporal cortex (statistical nonparametric mapping, p≤0.0004). No difference was observed in fast spindle parameters between the healthy and medicated comparison groups.
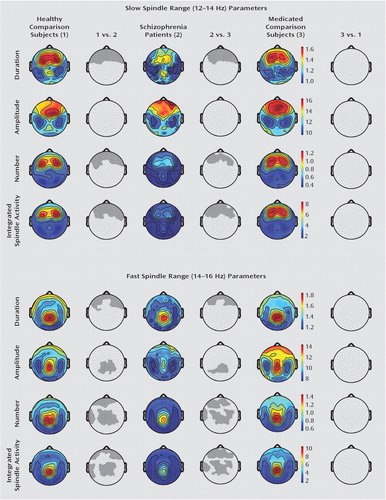
FIGURE 2. Slow and Fast Spindle Range Activity in Schizophrenia Patients, Nonschizophrenia Patients Receiving Antipsychotic Medication, and Healthy Subjectsa
a Deficits in slow (12–14 Hz) sleep spindle duration, number, and integrated activity in schizophrenia patients relative to both medicated (non-schizophrenia patients receiving antipsychotics) and healthy comparison subjects were established in a prefrontal region (statistical non-parametric mapping, p≤0.001). No significant reduction in slow spindle amplitude across the three groups as well as between healthy and medicated comparison subjects in the other slow spindle parameters was found. Deficits in fast (14–16 Hz) sleep spindle duration (prefrontal) and amplitude (centroparietal) as well as in number and integrated spindle activity (both prefrontal and centroparietal) were assessed in schizophrenia patients relative to both healthy and medicated comparison subjects (statistical nonparametric mapping, p≤0.001). Additionally, for fast spindle number and integrated spindle activity, a significant reduction in the left temporal cortex was established (statistical nonparametric mapping, p≤0.001). No significant reduction in any fast spindle parameter was found between healthy and medicated comparison subjects.
To determine the magnitude of spindle deficits in schizophrenia patients, Cohen's d, which measures effect size, was calculated for spindle parameters. Cohen's d analysis showed that prefrontal (for spindle duration), centroparietal (for spindle amplitude, number, and integrated activity), and left temporal (for spindle number and integrated activity) areas were the most affected in the schizophrenia group. Moreover, Cohen's d values ranged from an effect size equal to 1.4 for spindle amplitude to an effect size ≥2.21 for spindle number and integrated activity.
Slow Wave Analysis
In addition to slow wave activity (slow wave activity: 1–4.5 Hz), we computed slow wave incidence, negative peak amplitude, and down and up slopes. In a previous investigation, we demonstrated that each of these parameters represents different aspects of cortical synchronization during slow waves (11). The topography of these parameters showed similar antero-posterior gradients across groups, with clear peaks in prefrontal areas, where slow wave activity is usually maximal, and lowest values in posterior parietal and occipital areas (Figure 3). No significant difference for any slow wave parameter was found across groups (statistical nonparametric mapping, p≥0.2).
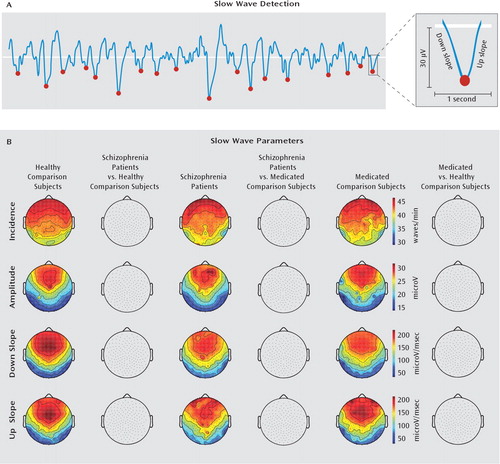
FIGURE 3. Slow Wave Parameters in Schizophrenia Patients, Nonschizophrenia Patients Receiving Antipsychotic Medication, and Healthy Subjectsa
a The images illustrate that slow wave parameters are not defective in schizophrenia. A) Slow waves occurring during whole-night non-REM sleep were detected using an automated algorithm, and several slow wave parameters, including incidence (waves/min), amplitude (microV), and down and up slopes (microV/msec), were measured. B) No significant difference (p<0.05) in the topography of each of these slow wave parameters was found across the three groups.
Cognitive Ability and Spindles
To establish possible correlations between general cognitive ability and spindles, schizophrenia patients (N=20), healthy subjects (N=19), and nonschizophrenia patients receiving antipsychotics (N=12) were administered a computerized version of the Ravens Progressive Matrices Test (14), which is widely used in research/clinical settings to measure intelligence (15). We found that schizophrenia patients (mean score: 36 [SD=9]) and medicated comparison subjects (mean score: 37.5 [SD=13]) had similar Ravens Progressive Matrices Test scores (p=0.94), but both had lower scores relative to healthy subjects (mean score: 44 [SD=9]). Moreover, Ravens Progressive Matrices Test scores of schizophrenia patients did not correlate with integrated spindle activity (r=0.18, p=0.42) or spindle number (r=0.17, p=0.48).
Clinical and Spindle Parameters in Schizophrenia Patients
We also performed multiple regression/correlation analyses between clinical characteristics and spindle parameters in the schizophrenia group (Table 2). Spindle number was inversely related to age (r=−0.33, p=0.021) and stereotyped thinking (N7) of PANSS negative symptoms (N7: r=−0.32, p=0.028). Moreover, both integrated spindle activity and spindle number were inversely related to positive symptoms of PANSS (integrated spindle activity: r=−0.40, p=0.005; spindle number: r=−0.37, p=0.01). Specifically, spindle number was correlated only with conceptual disorganization (P2) (P2: r=−0.34, p=0.03) and hallucinations (P3) (P3: r=−0.4, p=0.01), while integrated spindle activity was correlated exclusively with hallucinations (r=−0.48, p=0.002).
Parameter | Spindle Number | Integrated Spindle Activity | ||
---|---|---|---|---|
r | pa | r | pb | |
Duration of illness | −0.25 | 0.09 | −0.15 | 0.31 |
Hospitalizations | 0.09 | 0.53 | 0.18 | 0.21 |
Age | −0.33 | 0.02 | −0.09 | 0.51 |
Medication dose | −0.18 | 0.20 | −0.13 | 0.38 |
Positive symptoms | −0.40 | 0.005 | −0.37 | 0.01 |
Negative symptoms | −0.32 | 0.02 | −0.28 | 0.06 |
General symptoms | −0.20 | 0.17 | −0.19 | 0.20 |
TABLE 2. Correlations Between Clinical and Spindle Parameters in Schizophrenia Patients
Discussion
Schizophrenia patients had whole-night deficits in spindle amplitude, duration, number, and integrated activity. Spindle number and integrated spindle activity were the most affected (effect size: ≥2.21) and were inversely correlated with clinical symptoms. No spindle abnormalities were found in nonschizophrenia patients receiving antipsychotics, suggesting that medications are unlikely to be responsible for spindle deficits. In sharp contrast to changes in spindles, schizophrenia patients were no different from the two groups of comparison subjects in several slow wave parameters.
Slow Wave Findings in Schizophrenia
Slow waves are EEG manifestations of periods of synchronous firing and silence of cortical neurons at 1–4 Hz. Slow waves are cortically generated by intrinsic conductances and cortico-cortical connections, although thalamo-cortical neurons also contribute to the synchronous onset of cortical firing. Slow waves are prominent in non-REM stage 3, also known as slow wave sleep (10). While sleep architecture, including time spent in slow wave sleep, has been extensively explored in schizophrenia (16), slow wave activity, the integrated EEG power at 1–4 Hz, has been scantily investigated, with confiicting reports. Two studies reported negative findings (9, 17), and three studies found reduced slow wave activity in schizophrenia patients to be associated with marked slow wave sleep decrease (18–20). Slow wave sleep deficits have not been consistently found in schizophrenia patients (21), occur more often in institutionalized patients with profound cognitive impairment (22), and have been established in other psychiatric groups (23). We found no slow wave sleep or slow wave activity reduction in our schizophrenia group, which consisted of outpatient subjects with moderate cognitive deficits. Additionally, none of the other slow wave parameters measured (including incidence, amplitude, and up and down slopes), each reflecting different aspects of cortical synchronization, differed between schizophrenia patients and the two groups of comparison subjects. These findings confirm previous reports that slow wave deficits may involve just a subgroup of schizophrenia patients (21). The absence of slow wave impairments in schizophrenia also suggests that spindle deficits may not merely reflect reduced daytime experience, since slow waves are a highly reliable measure of daytime plastic changes. Specifically, a recent study in rats showed that more exploratory behavior during wakefulness determined increased slow wave activity during subsequent sleep and that slow wave activity correlated with levels of brain-derived neurotrophic factor, a molecular marker of plasticity (24). Moreover, numerous studies in humans reported that learning specific tasks increases slow wave activity in task-related cortical areas, while reducing inputs to specific cortical areas determines reduced slow wave activity in these areas (25).
Spindle activity deficits in schizophrenia
In the present as well as prior high-density EEG investigations, we found deficits in the spindle activity of schizophrenia patients. Only a handful of studies, based on a few EEG channels and a limited number of subjects, have previously investigated spindles in individuals with schizophrenia. One study reported higher spindle counts during the first non-REM episode in five schizophrenia patients relative to healthy comparison subjects (19). Schizophrenia patients also had a significant increase in stage 2 sleep, when spindles mostly occur, which is the likely explanation for the increased spindles incidence. Two other studies, of nine (26) and 11 (27) schizophrenia patients and healthy subjects found no difference in spindle parameters, but this may be a consequence of the limited number of channels (C3 and C4) and of the spindle range (slow: 12–14 Hz) analyzed. Indeed, in our previous high-density EEG study, we found that spindle deficits were localized medial to C3 and C4 (9), while in the present study we have established that slow spindle deficits were restricted to prefrontal regions.
Spindle deficits are present throughout the night
We previously reported reduced sleep spindles in schizophrenia patients during the first non-REM episode (9). In the present study, we have established that spindle deficits were present throughout the night and did not reflect differences in the time course of spindle activity between schizophrenia patients and healthy subjects (28).
Spindle deficits are unlikely a result of antipsychotics
Since studying large numbers of unmedicated schizophrenia patients presents ethical/logistical challenges, we recruited nonschizophrenia patients to evaluate the effects of antipsychotics on spindles. These patients showed no difference in spindle activity compared with healthy subjects, thus suggesting that antipsychotics unlikely explain the spindle deficits in schizophrenia patients. Few studies have investigated the effects of antipsychotics on sleep EEG in healthy subjects and schizophrenia patients. In healthy subjects, one study reported no difference in sleep EEG power after single doses of olanzapine (29). Another study found that single doses of haloperidol or risperi-done had no effects on sleep EEG, while olanzapine led to decreased high frequency (>10 Hz) power during the second and fourth sleep episodes (30). One study, where schizophrenia patients were randomly assigned to receive placebo or single-dose olanzapine, found that the olanzapine group showed reduced spindle density compared with baseline nights (31). Another study investigating long-term effects of olanzapine on sleep EEG in schizophrenia reported no difference in spindle power after 4 weeks of treatment (32). Altogether, these results suggest that olanzapine may be the antipsychotic medication that affects spindle activity in acute but not chronic doses. In the present study, only three schizophrenia patients were receiving olanzapine, and schizophrenia patients showed no correlation between duration/dosage of antipsychotics and spindle parameters.
Spindle deficits are unrelated to reduced general cognitive ability
We also investigated possible correlations between spindles and general cognitive ability assessed with Raven's Progressive Matrices Test, a test employed in research/ clinical settings to measure intelligence. Both schizophrenia patients and antipsychotic-medicated comparison subjects showed lower test scores than healthy subjects, yet only schizophrenia patients had spindle deficits. Moreover, these test scores of schizophrenia patients did not correlate with spindle parameters. These results suggest that spindle deficits in schizophrenia are unrelated to reduced general cognitive ability. However, these findings do not exclude possible correlations between spindle reduction and deficits in specific neurocognitive paradigms in schizophrenia. Certain neurocognitive paradigms of attention and memory have been examined as putative endophenotypes for schizophrenia (4). Furthermore, some studies have shown that spindles are associated with these cognitive functions (33). Thus, future investigations exploring the relationship between spindle deficits and specific neurocognitive deficits in schizophrenia may provide further insight into the neural circuits underlying such impairments.
Altered spindles suggest thalamic/thalamic reticular nucleus dysfunctions in schizophrenia
Animal studies have shown conclusively that the neuronal substrates of spindles involve a cortex-thalamic reticular nucleus-thalamus circuit. Specifically, it is well established that the thalamic reticular nucleus is the spindle pacemaker and that thalamic reticular nucleus/ thalamus circuits can generate spindles in isolation, although cortical inputs may contribute to initiate/ amplify spindle oscillations (8). Thus, spindle deficits in schizophrenia may result from impairments in either thalamic reticular nucleus/thalamus circuits or in corticothalamic afferents to these intrathalamic circuits. Of all spindle parameters, the number, which likely reflects the pacemaker activity of thalamic reticular nucleus/ thalamus circuits, was the most reduced in schizophrenia patients and correlated with both positive and negative symptoms. Moreover, our cohort of schizophrenia patients had no deficits in slow waves, cortically generated oscillations that are synchronized and propagated by cortico-thalamic connections. Altogether, these findings suggest that a dysfunction within thalamic reticular nucleus/thalamus circuits may be primarily responsible for spindle deficits in schizophrenia. However, corticothalamic afferents may also play a role, as suggested by neuroimaging (34), electrophysiological (35), and postmortem (36) studies reporting cortico-thalamic connectivity deficits in schizophrenia.
The thalamic reticular nucleus is uniquely placed between the thalamus and the cortex, since it receives excitatory afferents from both cortical and thalamic neurons and sends gamma aminobutyric acid (GABA)-ergic inhibitory projections to all thalamic nuclei. Cortical afferents to the thalamic reticular nucleus far outweigh thalamic projections, and recent findings revealed diffuse prefrontal projections to frontal as well as sensory thalamic reticular nucleus sectors, which may regulate the ability to perform tasks in an environment with competing sensory inputs (37). These data, together with evidence showing an involvement of the thalamus in cortico-cortical communication (38), suggest that thalamic reticular nucleus-thalamus circuits may play a critical role in cognitive functions, including working memory, language, and sensorimotor integration, which require a high degree of cortico-cortical coordination/synchronization and are defective in schizophrenia (15). The thalamic reticular nucleus is also a strategic hub where peripheral stimuli can be blocked or selectively enhanced on their way to the cortex. Recent evidence has shown directly that the thalamic reticular nucleus is implicated in sensory gating and attentional modulation. Intracranial recordings in primates showed that visual attention involves both increased activity in the lateral geniculate nucleus and reduced firing of the thalamic reticular nucleus, which sends inhibitory efferents to the lateral geniculate nucleus (39). Furthermore, pharmacologically induced reductions in thalamic reticular nucleus activity, established with intracellular recordings, induce P50 auditory gating deficits, reflected in increased test/conditioning auditory response ratios (40). Importantly, sensory gating and attention deficits occur in several groups of schizophrenia patients, including first-break and medication-naive patients (41, 42), and a recent functional magnetic resonance imaging (fMRI) study showed an increased homodynamic response in the thalamus, which was correlated with higher P50 response ratios in schizophrenia patients relative to healthy comparison subjects (43).
Given the limited research available, we can only speculate about the molecular mechanisms mediating thalamic reticular nucleus deficits in schizophrenia. Recent electro-physiological recordings in rats showed that during development, GABA currents induce depolarization in thalamic reticular nucleus neurons, which is responsible for the bursting activity observed during spindles (44). These findings suggest that GABA currents/receptors in the thalamic reticular nucleus play a critical role in the development of spindles and are consistent with the involvement of GABA deficits in the neurobiology of schizophrenia (45). The thalamic reticular nucleus also has a high number of alpha7 nicotinic receptors (46), which are associated with P50 deficits, learning disabilities, and hallucinations (41, 47–48). Based on these findings, it would be important to measure spindle activity and P50 in the same schizophrenia group to assess a possible common neurobiological basis for these deficits in schizophrenia.
Future studies will be needed to establish whether spindle deficits are present in other schizophrenia populations, including medication-naive and first-break patients. Such studies would help confirm spindle deficits at illness onset and in the absence of medication confounds. It will also be critical to employ compounds that selectively block/activate nicotinic, GABA, or N-methyl-D-aspartic acid receptors, which are localized on thalamic reticular nucleus neurons and are thought to be defective in schizophrenia. These studies could establish which drugs may ameliorate both symptoms and spindle activity in schizophrenia patients, thus providing further insight into which neurotransmitters, and neuronal pathways, are impaired in schizophrenia. Finally, transcranial magnetic stimulation in combination with high-density EEG and fMRI will be needed to evaluate the functioning of cortico-thalamic reticular nucleus-thalamic-cortical loops in schizophrenia (35).
1. : Family burden of schizophrenia and depressive illness: specifying the effects of ethnicity, gender and social ecology. Br J Psychiatry 1999; 174:31–38Crossref, Medline, Google Scholar
2. : Neurobiology of schizophrenia. Curr Opin Neurobiol 1997; 7:701–707Crossref, Medline, Google Scholar
3. : Schizophrenia genes, gene expression, and neuropathology: on the matter of their convergence. Mol Psychiatry 2005; 10:40–68Crossref, Medline, Google Scholar
4. : The consortium on the genetics of schizophrenia: neurocognitive endophenotypes. Schizophr Bull 2007; 33:49–68Crossref, Medline, Google Scholar
5. : Neurocognitive deficits and functional outcome in schizophrenia: Are we measuring the “right stuff”? Schizophr Bull 2000; 26:119–136Crossref, Medline, Google Scholar
6. : Schizophrenia endophenotypes as treatment targets. Expert Opin Ther Targets 2007; 11:1189–1206Crossref, Medline, Google Scholar
7. : Grouping of brain rhythms in corticothalamic systems. Neuroscience 2006; 137:1087–1106Crossref, Medline, Google Scholar
8. : The reticular nucleus revisited: intrinsic and network properties of a thalamic pacemaker. Prog Neurobiol 2005; 75:125–141Crossref, Medline, Google Scholar
9. : Reduced sleep spindle activity in schizophrenia patients. Am J Psychiatry 2007; 164:483–492Link, Google Scholar
10. : The visual scoring of sleep in adults. J Clin Sleep Med 2007; 3:121–131Medline, Google Scholar
11. : Sleep homeostasis and cortical synchronization, III: a high-density EEG study of sleep slow waves in humans. Sleep 2007; 30:1643–1657Crossref, Medline, Google Scholar
12. : Nonparametric permutation tests for functional neuroimaging: a primer with examples. Hum Brain Mapp 2002; 15:1–25Crossref, Medline, Google Scholar
13. : Statistical Power Analysis for the Behavioral Sciences. Hillsdale, NJ, Erlbaum, 1988Google Scholar
14. : The Raven's Progressive Matrices: change and stability over culture and time. Cogn Psychol 2000; 41:1–48Crossref, Medline, Google Scholar
15. : Computerized neurocognitive scanning, II: the profile of schizophrenia. Neuropsychopharmacology 2001; 25:777–788Crossref, Medline, Google Scholar
16. : Sleep disturbance in schizophrenia. Int Rev Psychiatry 2005; 17:247–253Crossref, Medline, Google Scholar
17. : High frequency EEG activity during sleep: characteristics in schizophrenia and depression. Clin EEG Neurosci 2005; 36:25–35Crossref, Medline, Google Scholar
18. : Delta power in sleep in relation to neuropsychological performance in healthy subjects and schizophrenia patients. J Neuropsychiatry Clin Neurosci 2006; 18:529–535Crossref, Medline, Google Scholar
19. : Further evidence of abnormal non-rapid-eye-movement sleep in schizophrenia. Arch Gen Psychiatry 1985; 42:797–802Crossref, Medline, Google Scholar
20. : Delta sleep deficits in schizophrenia: evidence from automated analyses of sleep data. Arch Gen Psychiatry 1998; 55:443–448Crossref, Medline, Google Scholar
21. : Does abnormal sleep impair memory consolidation in schizophrenia? Front Hum Neurosci 2009; 3:21Crossref, Medline, Google Scholar
22. : Clinical significance of sleep EEG abnormalities in chronic schizophrenia. Schizophr Res 2006; 82:251–260Crossref, Medline, Google Scholar
23. : Sleep in mood disorders. Psychiatr Clin North Am 2006; 29:1009–1032Crossref, Medline, Google Scholar
24. : Exploratory behavior, cortical BDNF expression, and sleep homeostasis. Sleep 2007; 30:129–139Crossref, Medline, Google Scholar
25. : Slow waves, synaptic plasticity and information processing: insights from transcranial magnetic stimulation and high-density EEG experiments. Eur J Neurosci 2009; 29:1761–1770Crossref, Medline, Google Scholar
26. : Circadian and sleep-related endocrine rhythms in schizophrenia. Arch Gen Psychiatry 1991; 48:348–356Crossref, Medline, Google Scholar
27. : Sleep architecture and its clinical correlates in first episode and neuroleptic-naive patients with schizophrenia. Schizophr Res 2003; 62:147–153Crossref, Medline, Google Scholar
28. : Sleep spindles: an overview. Sleep Med Rev 2003; 7:423–440Crossref, Medline, Google Scholar
29. : Effect of a single-dose of olanzapine on sleep in healthy females and males. Int Clin Psycho-pharmacol 2002; 17:177–184Crossref, Medline, Google Scholar
30. : Effects of olanzapine, risperidone and haloperidol on sleep after a single oral morning dose in healthy volunteers. Psychopharmacology (Berl) 2007; 190:507–516Crossref, Medline, Google Scholar
31. : Effects of olanzapine on slow wave sleep, sleep spindles and sleep-related memory consolidation in schizophrenia. Pharmacopsychiatry 2008; 41:92–99Crossref, Medline, Google Scholar
32. : Sub-chronic effects of olanzapine on sleep EEG in schizophrenic patients with predominantly negative symptoms. Pharmaco-psychiatry 2004; 37:157–162Crossref, Medline, Google Scholar
33. : Sleep spindles and learning potential. Behav Neurosci 2007; 121:1–10Crossref, Medline, Google Scholar
34. : Basal ganglia-thalamocortical circuitry disruptions in schizophrenia during delayed response tasks. Biol Psychiatry 2006; 60:235–241Crossref, Medline, Google Scholar
35. : Reduced evoked gamma oscillations in the frontal cortex in schizophrenia patients: a TMS/EEG study. Am J Psychiatry 2008; 165:996–1005Link, Google Scholar
36. : Maldistribution of interstitial neurons in prefrontal white matter of the brains of schizophrenic patients. Arch Gen Psychiatry 1996; 53:425–436Crossref, Medline, Google Scholar
37. : Circuits formultisensory integration and attentional modulation through the prefrontal cortex and the thalamic reticular nucleus in primates. Rev Neurosci 2007; 18:417–438Crossref, Medline, Google Scholar
38. : Thalamic relays and cortical functioning. Prog Brain Res 2005; 149:107–126Crossref, Medline, Google Scholar
39. : Guarding the gateway to cortex with attention in visual thalamus. Nature 2008; 456:391–394Crossref, Medline, Google Scholar
40. : Auditory sensory gating in hippocampus and reticular thalamic neurons in anesthetized rats. Biol Psychiatry 2003; 53:244–253Crossref, Medline, Google Scholar
41. : The genetics of sensory gating deficits in schizophrenia. Curr Psychiatry Rep 2003; 5:155–161Crossref, Medline, Google Scholar
42. : Early biomarkers of psychosis. Dialogues Clin Neurosci 2005; 7:17–29Medline, Google Scholar
43. : Increased hemodynamic response in the hippocampus, thalamus and prefrontal cortex during abnormal sensory gating in schizophrenia. Schizophr Res 2007; 92:262–272Crossref, Medline, Google Scholar
44. : Giant spontaneous depolarizing potentials in the developing thalamic reticular nucleus. J Neurophysiol 2007; 97:2364–2372Crossref, Medline, Google Scholar
45. : The neuronal pathology of schizophrenia: molecules and mechanisms. Biochem Soc Trans 2007; 35(pt 2):433–436Crossref, Medline, Google Scholar
46. : Alpha-7 nicotinic receptor agonists: potential new candidates for the treatment of schizophrenia. Psychopharmacology (Berl) 2004; 174:54–64Crossref, Medline, Google Scholar
47. : The construct of attention in schizophrenia. Biol Psychiatry 2008; 64:34–39Crossref, Medline, Google Scholar
48. : Neuronal nicotinic receptors in dementia with Lewy bodies and schizophrenia: alpha-bungarotoxin and nicotine binding in the thalamus. J Neurochem 1999; 73:1590–1597Crossref, Medline, Google Scholar