Abnormal fMRI Response of the Dorsolateral Prefrontal Cortex in Cognitively Intact Siblings of Patients With Schizophrenia
Abstract
OBJECTIVE: The identification of neurobiological intermediate phenotypes may hasten the search for susceptibility genes in complex psychiatric disorders such as schizophrenia. Earlier family studies have suggested that deficits in executive cognition and working memory may be related to genetic susceptibility for schizophrenia, but the biological basis for this behavioral phenotype has not been identified. METHOD: The authors used functional magnetic resonance imaging (fMRI) during performance of the N-back working memory task to assess working memory-related cortical physiology in nonschizophrenic, cognitively intact siblings of patients with schizophrenia. They compared 23 unaffected siblings of schizophrenic patients to 18 matched comparison subjects. As a planned replication, they studied another 25 unaffected siblings and 15 comparison subjects. RESULTS: In both cohorts, there were no group differences in working memory performance. Nevertheless, both groups of siblings showed an exaggerated physiological response in the right dorsolateral prefrontal cortex that was qualitatively similar to results of earlier fMRI studies of patients with schizophrenia. CONCLUSIONS: These fMRI data provide direct evidence of a primary physiological abnormality in dorsolateral prefrontal cortex function in individuals at greater genetic risk for schizophrenia, even in the absence of a manifest cognitive abnormality. This exaggerated fMRI response implicates inefficient processing of memory information at the level of intrinsic prefrontal circuitry, similar to earlier findings in patients with schizophrenia. These data predict that inheritance of alleles that contribute to inefficient prefrontal information processing will increase risk for schizophrenia.
Schizophrenia is a highly heritable condition, but genetic susceptibility is complex and thought to arise from the combined effects of multiple susceptibility alleles and environmental factors. This has complicated the search for these susceptibility genes with traditional linkage approaches (1, 2). An alternative approach is based on the identification of so-called intermediate phenotypes (e.g., deficits in cognition [3] or abnormalities in evoked electrophysiological potentials [4]) that are identified in patients with schizophrenia and in their unaffected relatives. It is presumed that these phenotypes reflect a more predictable effect of susceptibility genes (5, 6). In general, the search for intermediate phenotypes relies on the fact that siblings, even when unaffected by the full-blown illness under study, share on average 50% of their ill siblings’ alleles. Thus, to the extent that susceptibility alleles are related to a putative intermediate phenotype, siblings express that phenotype at a frequency higher than the general population (i.e., have a greater relative risk) (7, 8). Analogous approaches to phenotypic reduction and to gene discovery have been used in other complex genetic disorders, such as colon cancer, hypertension, and diabetes (9).
Deficits in cortical information processing have been especially attractive intermediate phenotypes related to schizophrenia as they are stable and cardinal features of the illness and are also found with greater frequency in healthy relatives (10–16). Information processing refers to the flow of information through various brain circuits during simple (e.g., sensory perception) and complex (e.g., problem solving) cognitive processes. In a more narrow sense, information processing also connotes the synaptic architecture and functional characteristics of the neuronal assemblies handling this flow of information. Deficits of various aspects of information processing are frequently found in schizophrenia and include abnormalities of sensory gating measures, such as the P50 evoked potential (15), and impairment of cognitive tasks, such as those involving working memory (17–20) and executive cognition (e.g., as measured by the Wisconsin Card Sorting Test) (21, 22). Abnormal function of the dorsolateral prefrontal cortex has been prominently discussed as a source of these cognitive deficits (21, 23).
Deficits in working memory and executive cognition also appear to be related to genetic risk for schizophrenia. For example, Goldberg et al. (24) examined working memory in monozygotic twins who were discordant for schizophrenia and in normal monozygotic twins. Subtle working memory deficits were found even in the unaffected twins that implicated a genetic component to working memory dysfunction that was transmitted independently from manifest schizophrenia. More recently, Cannon et al. (25) examined the heritability of a variety of neuropsychological deficits in 18 monozygotic and 30 dizygotic twin pairs who were discordant for schizophrenia and found strong evidence for genetic loading in spatial working memory deficits. Analogous results have been reported in studies of nontwin siblings of patients with schizophrenia (13, 26). The presence of greater concordance for working memory deficits than for the diagnosis of schizophrenia in sibships raises some important issues. First, it suggests that susceptibility genes for schizophrenia may affect the biological mechanisms of working memory and executive cognition. Second, genetic analyses solely reliant on manifest schizophrenia may fail to identify some carriers of susceptibility alleles who manifest an intermediate phenotype related to prefrontal dysfunction. More important, if we observe greater concordance in families for working memory deficits than for manifest schizophrenia, we might imagine even greater concordance for phenotypes based on the abnormal physiology underlying working memory deficits. This is assumed because behavioral deficits presumably result from a physiological abnormality, while a physiological abnormality does not necessarily predict a behavioral deficit, as cognitive compensation may occur in otherwise intact individuals. Thus, it might be possible to get even closer to the biological effects of susceptibility genes in unaffected siblings by targeting primary physiological abnormalities independent of the presence of behavioral deficits.
Functional neuroimaging has recently identified a physiological abnormality in patients that may have these characteristics. Using blood-oxygenation-level-dependent (BOLD) functional magnetic resonance imaging (fMRI), two laboratories have demonstrated that schizophrenia patients who perform nearly as well as comparison subjects on certain working memory tasks manifest an exaggerated activation response at the dorsolateral prefrontal cortex (27–29). Although the mechanism of this abnormal fMRI response remains unclear, and it is likely related to the particulars of the experimental design, we have described it empirically as functional inefficiency of the dorsolateral prefrontal cortex based on the notion that the increase in the fMRI response (and presumably the neuronal activity underlying it) does not produce greater accuracy. However, this exaggerated response of the dorsolateral prefrontal cortex is not a sine qua non of the dorsolateral prefrontal cortex in schizophrenia, as other physiological abnormalities have been described (e.g., lower response in the dorsolateral prefrontal cortex assessed under different conditions with functional neuroimaging [21, 22, 30–41]). Notwithstanding uncertainty about its mechanism, the inefficiency response of the dorsolateral prefrontal cortex is particularly appealing for study as a potential genetic susceptibility trait for several reasons including the fact that 1) it is not easily correlated with abnormal behavior per se, such as reduced performance, attention, or motivation (as are underactivation responses), and 2) a functional polymorphism in the gene for catechol O-methyltransferase (COMT) that has been associated with schizophrenia (42–44) also has been associated with our fMRI measure of inefficiency in the dorsolateral prefrontal cortex (45).
We hypothesized, therefore, that the inefficiency response of the dorsolateral prefrontal cortex is a biological trait related to genetic risk for schizophrenia and that as a manifestation of abnormal information processing in the circuitry of the dorsolateral prefrontal cortex, it would be apparent even if compensation could be made at the manifest behavioral level. To test this hypothesis, we used the same fMRI and working memory protocol as previously described (29, 34, 46) to study cognitively unimpaired and clinically unaffected siblings of schizophrenic patients in relation to healthy comparison subjects. We predicted that physiological inefficiency of the dorsolateral prefrontal cortex would be found in unaffected siblings, even without deficits in task performance. We studied two independent cohorts of unaffected siblings with no apparent working memory deficits at the level of task performance. During the interval between the collection of these two data sets, considerable improvements in fMRI methods were made. Regardless, we found complementary evidence in both cohorts that an abnormal fMRI response of the dorsolateral prefrontal cortex during working memory challenges may be an intermediate phenotype associated with genetic risk for schizophrenia.
Method
Subjects and Experimental Protocol
We performed two studies in two independent groups—an initial investigation followed by a planned replication. In the first study, we compared 38 unaffected siblings of schizophrenic patients to 32 healthy comparison subjects using the N-back working memory task. fMRI data from 18 healthy comparison subjects were reported previously (29). In the second study, we compared fMRI data from 30 unaffected siblings and 23 healthy comparison subjects. The details of recruitment of patients, their siblings, and healthy comparison subjects as part of the Clinical Brain Disorders Branch Sibling Study (Protocol 95-M-0150) are described elsewhere (16). Briefly, patients were recruited from local and national sources advertised in print, by word of mouth, and on the Internet. Probands with diagnoses of schizophrenia and schizoaffective disorder were selected. All siblings of suitable probands were contacted and invited to participate. Healthy volunteers were recruited from the National Institutes of Health Clinical Research Volunteer Program and were screened in an identical fashion to patients and siblings. This study was approved by the institutional review board of the intramural program of the National Institute of Mental Health. All subjects gave written informed consent before participation.
Healthy siblings of schizophrenic patients (both cohorts) and healthy volunteers were given the Structured Clinical Interview for DSM-IV (SCID) (47) to determine the presence of any psychiatric illnesses, a neurological examination, a battery of neuropsychological tests, an EEG, and a screening MRI examination (16). Siblings were included only if they did not have schizophrenia or a schizophrenia spectrum disorder (e.g., schizoaffective disorder, schizotypal and schizoid personality disorders)—hereafter referred to as “unaffected siblings.” Additional exclusion criteria included the inability to give informed consent, an IQ below 70, a history of substance abuse within the past 6 months, a history of significant neurological illness, and any focal abnormalities found by EEG or MRI. The healthy comparison subjects were screened by using the same criteria. The comparison subjects were matched to the siblings for age, gender, years of education, handedness, and socioeconomic status as closely as possible.
After the fMRI data collection, our most important inclusion criterion was fMRI data quality, as has been discussed elsewhere in greater detail (35, 48). From the first study, 15 siblings and 14 comparison subjects were excluded for insufficient data quality, leaving a final cohort of 23 unaffected siblings and 18 healthy comparison subjects (Table 1). In the second study, five siblings and eight comparison subjects were excluded because of insufficient data quality, leaving a final second cohort of 25 siblings and 15 comparison subjects (Table 1). While comparison subjects and siblings were screened to exclude schizophrenia spectrum diagnoses, four unaffected siblings had a history of major depression. Overall, 78% of the siblings from these families were successfully recruited. In general, more than one sibling per proband was recruited wherever possible; however, only one sibling per proband was included in the two studies reported herein. The probands of these siblings were composed of 18 patients with schizophrenia and five patients with schizoaffective disorder, depressed type, with the following demographic characteristics: age 39 years (SD=10), 20 of 23 were men, duration of illness=16 years (SD=10), 18 of 23 treated with atypical antipsychotics (chlorpromazine-equivalent dose=584 mg, SD=383), and 14 years of education (SD=2). In the second study, four of the unaffected siblings had a history of major depression. Overall, 87% of the siblings from these families were successfully recruited. The probands of these siblings were composed of 22 patients with schizophrenia and three patients with schizoaffective disorder with the following demographic characteristics: age 38 years (SD=7), 22 of 25 were men, duration of illness=17 years (SD=9), 14 of 25 treated with atypical antipsychotics (chlorpromazine-equivalent dose=337 mg, SD=154), and 14 years of education (SD=2). Attrition was not selective, and all data-quality checks were performed blind to subject identity and diagnosis. The demographic characteristics were similar between those included and those excluded. Specifically, IQ, score on the Wide Range Achievement Test, Revised (WRAT-R) (51), age, education, and family socioeconomic status were not different in the first study. The only significant difference in the second study was that IQ was slightly higher for the siblings included (IQ=110) than those excluded (IQ=106) (t=2.1, df=28, p=0.04). N-back performance did not differ between those included and those excluded in either study. All subjects included in these analyses were of European American ancestry. The unaffected siblings were not different from the comparison subjects in global cognitive function (comparison subjects’ mean IQ=105.9, SD=15.8, versus siblings’ mean IQ=108.3, SD=13.0; comparison subjects’ mean WRAT-R score=108, SD=7, versus siblings’ mean WRAT-R score=109, SD=9).
All subjects underwent a training session immediately before fMRI data acquisition to practice the N-back task as described previously (29, 35, 46). Designed to force subjects to constantly update their mental set while minimizing interference from incoming stimuli, our version of the N-back task consisted of continual presentation of visual stimuli (the numbers 1–4 appeared randomly every 1.8 seconds for 500 msec) at set locations at the points of a diamond-shaped box (Figure 1). In contrast to other versions of the N-back task, every number was both a probe and a target (i.e., a 100% target). Instructions displayed above the diamond informed the subjects to recall the stimulus seen “N” previously. The stimuli were generated by a standard desktop computer running in-house software (by R. Coppola) and presented by means of a fiber-optic goggle system (Resonance Technology, Van Nuys, Calif.). Responses were recorded by means of a fiber-optic response box with buttons arrayed in the same configuration as the stimuli presented on the screen and relayed back to the computer for tabulation of performance accuracy. The original N-back program used in the first study only recorded accuracy; however, reaction time was added for the second study. Working memory load was varied across the following range: 0 back, 1 back, and 2 back in the first study and between 0 back and 2 back in the second study. The different levels of working memory load (0 back, 1 back, and 2 back) entailed delays of 0, 1.8, and 3.6 seconds, respectively, between the probe and the target. Before beginning the fMRI scanning session, all of the subjects were trained to the point at which their performance (accuracy) for each level of the task (0 back, 1 back, and 2 back) remained constant. We found no significant changes in N-back performance over the duration of a typical fMRI scanning session beyond this point (35).
Demographic characteristics were compared between the siblings and the comparison subjects in the following manner: gender and handedness were compared between groups by using chi-square, while age, years of education, and socioeconomic status were compared between groups by using two-tailed t tests (STATISTICA, StatSoft, Inc., Tulsa, Okla.). Performance data were analyzed as follows: between-group comparisons of performance (both studies) and reaction time (second study only) were made by using repeated-measures analysis of variance (ANOVA) with diagnosis as the between-group and working memory load as the within-group factors, followed by post hoc planned comparisons (STATISTICA, StatSoft, Inc., Tulsa, Okla.).
Study 1: fMRI Data Acquisition
fMRI studies were performed on a standard 1.5-T General Electric Signa Scanner (Milwaukee) outfitted with a combined radio frequency and gradient insert coil (Medical Advances, Milwaukee). Echo planar imaging BOLD fMRI data (52) were acquired as described previously (interleaved, TE=60 msec, whole-brain TR=4 seconds, voxel dimensions=3.75 mm isotropic) (53). High-resolution T1-weighted spin-echo whole-brain anatomical images (TE=10 msec, TR=500 msec, 3.75 mm isotropic voxels) were coregistered to the fMRI data for localization. In the first study, working memory load was pseudorandomized between 0-back, 1-back, and 2-back tasks and interspersed with an eyes-open fixation condition consisting of a blank diamond (rest) (Figure 1). The rate of presentation was held constant throughout at approximately every 1800 msec. Task conditions lasted 20 seconds, with eight conditions per block. Nine such blocks were collected (35). Ninety whole-brain fMRI volumes were obtained for each working memory load (i.e., 180 whole-brain fMRI volumes for a comparison of 0-back to 2-back tasks).
Study 2: fMRI Data Acquisition
In the second study, the subjects received the same instructions and prescanning routine. fMRI was performed on the same 1.5-T General Electric Signa Scanner (Milwaukee) by using a standard head coil and a fast spiral sequence (interleaved, TE=24 msec, whole-brain TR=2000 msec, voxel size=3.75 mm isotropic) (54). As during echo-planar imaging data collection, whole-brain anatomical images were acquired for localization. The rate of presentation of stimuli and all other N-back characteristics were the same as those previously noted. In the second study, however, we used a simpler block design in which one block consisted of eight alternating 0-back and 2-back task conditions. Task conditions lasted for 16 seconds, with 16 conditions per block. Each block lasted 256 seconds, with 16-second conditions alternating between 0-back and 2-back. A total of 128 whole-brain fMRI volumes were obtained. Technical advances introduced between the first and second studies (54) allowed for a simpler design that produced a similar number of whole-brain volumes for each individual (180 versus 128, respectively) and reduced the time spent in the magnet for all subjects.
fMRI Data Analysis
For both studies, fMRI data processing began with registration of whole-brain fMRI volumes by using a sinc interpolation (55) to the initial fMRI brain volume of a block. These whole-brain volumes were then transformed into standard space (55–58). Voxel-wise signal intensities were ratio normalized to the whole-brain mean and then detrended in a linear fashion with the baseline at each voxel set to 1,000. To control for interindividual variance in regional anatomy, data were smoothed with a Gaussian filter (10×10×10 mm) in the same manner as has been applied to previous patient data sets (29, 35). We analyzed these data using statistical parametric mapping (SPM 99; http://www.fil.ion.ucl.ac.uk/apm) (59). In both studies, fMRI data were analyzed as time series modeled by a sine wave shifted by an estimate of the hemodynamic response. Individual subject maps were created by using one-sample t tests. The resultant contrast images were then entered into second-level (random effects) analyses for each data set separately, consisting of ANOVAs comparing siblings and comparison subjects, followed by analyses of covariance (ANCOVAs) examining the effects of age, education, and gender as determined by the demographic differences between groups. Finally, to address activation commonalities between data sets, we used the statistical parametric mapping results from the first study to create a mask that limited the analysis to the dorsolateral prefrontal cortex regions showing a significant group difference between unaffected siblings and comparison subjects. Statistically significant group differences (Table 2) were reported as voxel-intensity z values. For anatomical localization, statistical maxima of activation were converted to conform to the standard space of Talairach and Tournoux (60). For the first study, we chose a statistical threshold of p<0.01 (uncorrected) with a minimum cluster size of eight voxels. Because the second study was a planned replication, we adopted a more liberal threshold of p<0.05 and a minimum cluster size of eight voxels.
Results
Demographic and Behavioral Data
In the first study, the groups did not differ significantly in age, handedness, or socioeconomic status (Table 1). Siblings in this study had significantly fewer years of education but did not differ in socioeconomic status, suggesting that siblings and comparison subjects were reasonably matched for intellectual ability. In addition, the male/female ratio differed between the groups, with more female siblings included in this cohort. Although the siblings had significantly fewer years of education and differed in the ratio of male/female subjects (χ2=5.10, df=1, p<0.05) in study 1, this was not the case in the replication study. Nonetheless, ANCOVAs with age, years of education, and gender as confounds failed to change these results. In the second study, the siblings did not differ from the comparison subjects in years of education, socioeconomic status, or ratio of males/females. However, there was a small but significant difference in age (t=3.10, df=38, p<0.01) in the second study (Table 1). Again, ANCOVA with age failed to change the results. In summary, the siblings and comparison subjects were reasonably well matched. The most consistent difference between the comparison subjects and siblings was that the comparison subjects were slightly younger in study 1 (t=1.95, df=39, p=0.06) and significantly different in study 2. In summary, none of these demographic differences accounted for the findings as revealed by additional ANCOVA.
In the first study, the unaffected siblings of patients with schizophrenia were indistinguishable from the healthy comparison subjects in terms of N-back working memory accuracy (main effect of diagnosis: F=0.13, df=1, 39, p=0.71; main effect of load: F=23.0, df=2, 78, p<0.001; interaction of diagnosis by load: F=0.02, df=2, 78, p=0.94) (Table 1). Compared to working memory accuracy on the 0-back task, performance was significantly worse, for both siblings and comparison subjects, on the 1-back and 2-back tasks (F=24.23, df=2, 80, p<0.01). N-back performance was not correlated with age, gender, or years of education. In the replication study, sibling performance was again indistinguishable from that of the comparison subjects for both the 0-back and 2-back tasks (main effect of diagnosis: F=0.28, df=1, 38, p=0.59; main effect of load: F=14.14, df=1, 36, p<0.001; interaction of diagnosis by load: F=0.01, df=1, 38, p=0.91) (Table 1). Again, compared to 0-back working memory accuracy, performance was worse for both the siblings and comparison subjects in the 2-back task. There also were no significant differences in 2-back reaction time between the siblings and comparison subjects (main effect of diagnosis: F=0.19, df=1, 36, p=0.66) (Table 1). N-back performance was significantly correlated with age (r=–0.37, p=0.02) but not with gender or education.
fMRI Data
In spite of similarities in working memory behavioral measures, the unaffected siblings and comparison subjects differed in their respective fMRI response to varying working memory load in both studies. There were no significant group differences in 1-back task performance in study 1. However, siblings in 2-back performance were hyperresponsive in a number of key working memory-related cortical regions, most notably the right dorsolateral prefrontal cortex (Brodmann’s areas 9/10, 46) (Table 2, Figure 2, top), as found previously in schizophrenic patients (29). In addition, we found an exaggerated response in the bilateral inferior frontal gyrus (Brodmann’s area 44/45), bilateral anterior cingulate (Brodmann’s area 24/32), bilateral inferior parietal lobule (Brodmann’s area 40), bilateral precuneus (Brodmann’s area 7), right medial frontal gyrus (Brodmann’s area 6), and right middle temporal gyrus (Brodmann’s area 21/39). The healthy comparison subjects had a greater fMRI response in only one prefrontal region—the left medial frontal gyrus (Brodmann’s area 10), a region not significantly related to working memory function (46) (Table 2). The healthy comparison subjects were also more active in the right hippocampus, left precuneus (Brodmann’s area 7), bilateral thalamus, right superior temporal gyrus (Brodmann’s area 22/42), and several foci within the bilateral cerebellum. Greater activation in the cerebellum, hippocampus, and superior temporal gyrus was in close proximity to areas in which the comparison subjects were more active than the schizophrenia patients in our previous study (29).
In the second study, we identified the same regional difference in the right dorsolateral prefrontal cortex (Brodmann’s area 9/10) between the siblings and the comparison subjects (Table 2, Figure 2, bottom). Areas of overactivation seen in the second study that did not appear in the first study included the right inferior frontal gyrus (47), posterior cingulate (Brodmann’s area 31), fusiform gyrus (Brodmann’s area 19), and right thalamus. The only areas of overlap were the right dorsolateral prefrontal cortex and the left inferior parietal lobule (Brodmann’s area 40). This was confirmed after masking the analysis with the results from study 1, revealing two areas of common activation in the right dorsolateral prefrontal cortex: the middle frontal gyrus (Brodmann’s area 9) (x=48, y=17, z=29) (z=2.17) and the middle frontal gyrus (Brodmann’s area 46) (x=40, y=42, z=15) (z=1.80) (Figure 2, right). At the same time, the healthy comparison subjects showed greater activation in the bilateral premotor cortex (Brodmann’s area 6), left medial frontal gyrus (Brodmann’s area 10), left parietal cortex (Brodmann’s areas 7, 40), bilateral anterior cingulate (Brodmann’s area 24/32), bilateral posterior cingulate (Brodmann’s area 31), bilateral fusiform gyrus (Brodmann’s area 18/37), left thalamus, right hippocampus, and cerebellum. Thus, the areas commonly overactivated in the healthy comparison subjects included the medial frontal gyrus (Brodmann’s area 10), left precuneus (Brodmann’s area 7), posterior cingulate, thalamus, and right hippocampus. In summary, in two separate cohorts, the unaffected siblings of schizophrenia patients showed greater activation in the right dorsolateral prefrontal cortex and left parietal lobule (both areas associated with working memory function), while the healthy comparison subjects were more active in areas outside of the typical working memory network.
Discussion
In two separate cohorts of cognitively intact and clinically unaffected siblings of schizophrenia patients, we identified an abnormal fMRI response in the dorsolateral prefrontal cortex characterized as an exaggerated or inefficient response of the dorsolateral prefrontal cortex during working memory activation. This abnormality would not have been predicted by performance on the working memory task, since the siblings did not perform abnormally, in terms of either response accuracy or response speed. This quantitative difference in activation suggested a qualitative difference in information processing within the dorsolateral prefrontal cortex that was not apparent at the level of manifest behavior. Overactivation of the right dorsolateral prefrontal cortex was the only finding consistently present in both sibling cohorts and in our earlier comparison of schizophrenia patients to comparison subjects (29). A similar exaggerated response has also been observed by other investigators using a different working memory paradigm, although in the opposite hemisphere (27, 28). As such, dorsolateral prefrontal cortex inefficiency is a replicable and abnormal physiological characteristic of information processing during working memory activation found in both schizophrenia patients and their unaffected siblings. While earlier twin studies had demonstrated a heritable component to working memory deficits in schizophrenia that indirectly implicated malfunction of the dorsolateral prefrontal cortex (12, 25), the current data provide direct evidence of a physiological abnormality in information processing by the dorsolateral prefrontal cortex that may contribute to—but is transmitted independently of—manifest schizophrenic illness.
While the dorsolateral prefrontal cortex is not the only region involved in processing working memory or activated during performance of working memory tasks, differences between siblings and comparison subjects in other regions outside of the right dorsolateral prefrontal cortex did not show a consistent pattern between studies. For example, unaffected siblings showed an inefficiency response in the inferior parietal lobule (right Brodmann’s area 40) and in the anterior cingulate that was not replicated in the second study. Within the working memory network, parietal and cingulate cortices are important nodes that have been found to be functionally abnormal in some studies of patients with schizophrenia (41, 61). However, other data suggest that abnormal neuronal function of the dorsolateral prefrontal cortex may be a primary deficit that drives more distributed abnormalities within the working memory network (62). In two studies of N-acetylaspartate, an intracellular neurochemical marker of neuronal integrity assessed with proton magnetic resonance spectroscopic imaging, only dorsolateral prefrontal cortex measures predicted activation within the working memory network (29, 63).
Several caveats to the use of dorsolateral prefrontal cortex inefficiency as an intermediate phenotype are worth noting. First, because a cognitive probe like the N-back task evokes a distributed cortical network, the interpretation of abnormal activation in any single node of this network is somewhat unclear. Abnormal activation may arise as a direct consequence of pathology in this region but may also represent the down- or upstream effects of abnormalities in other working memory nodes. Evidence against this interpretation comes from our previous demonstrations that dorsolateral prefrontal cortex neuropathology (i.e., N-acetylaspartate measures) selectively and exclusively predicts activation both in the dorsolateral prefrontal cortex and in other nodes of the working memory network in patients with schizophrenia (29, 63). While it might be desirable to map abnormal physiology with a more regionally selective task, this may be difficult in the dorsolateral prefrontal/supramodal cortex, which participates in a variety of cortical functions and exhibits complex inputs from—and projections to—many cortical and subcortical locales. We also cannot conclusively exclude a psychological explanation for our results (e.g., a difference in cognitive strategy in siblings), but this explanation seems superficial given the normal performance of all subjects and the subliminal nature of fMRI responses. More likely, the fMRI data suggest that at some level (e.g., synaptic architecture and functional circuitry) the cellular strategy for processing working memory information is abnormal in healthy siblings of patients with schizophrenia.
There were some demographic differences between our two study groups (although none were consistent in both cohorts) that could affect our findings. In the first cohort, the siblings had slightly fewer years of education than the comparison subjects. While general intelligence has been correlated with working memory capacity in healthy subjects, we failed to find a difference in accuracy between these two groups. Furthermore, years of education did not differ in the replication group. The unaffected siblings in the second cohort were slightly but significantly older than the comparison subjects, and the siblings were slightly older in the first study. Imaging studies have failed to identify a consistent pattern of activation changes associated with aging (finding both greater and less activation during cognitive activation in elderly than in younger subjects) (64). Rypma and D’Esposito (65) found age-related fMRI differences during working memory activation; however, older subjects activated the dorsolateral prefrontal cortex less than younger subjects during memory retrieval, which is unlikely to explain greater activation in siblings. Most convincingly, ANCOVAs using age, gender, and years of education failed to alter our results. Given the uncorrected thresholds used in both studies, the potential for false positive results arises. However, such concerns are diminished by the replication of dorsolateral prefrontal cortex findings across studies.
Another caveat is that the ultimate usefulness of the intermediate phenotype approach in the genetics of complex illnesses will depend upon the assumption that intermediate phenotypes arise with simpler, ideally Mendelian genetic architecture. However, a complex cognitive function like working memory that involves several cortical regions and multiple-component cognitive functions (e.g., maintenance, retrieval, error detection, minimization of interference) may possess as complex a genetic architecture as schizophrenia itself. However, strong support for the assumption that cognitively based intermediate phenotypes will simplify the gene search comes from two recent studies. First, Freedman et al. (15) demonstrated linkage between an abnormal evoked P50 response that was associated with schizophrenia and a locus on chromosome 15. This finding has led to a refined search for chromosome 15q and identification of a likely candidate gene (66). Second, Egan et al. (45) found that inheritance of the valine allele of the COMT gene is associated with schizophrenia, relatively abnormal executive cognition, and dorsolateral prefrontal cortex physiological efficiency in both healthy and ill populations. A single nucleotide polymorphism in COMT (Val 108/Met 158) confers a fourfold difference in enzymatic activity (67). Using this N-back fMRI paradigm with equal performance across genotypes, they found a dramatic effect on prefrontal efficiency with Met-Met individuals most efficient (with least activation), heterozygous Val-Met individuals intermediate in efficiency (with greater activation), and homozygous Val-Val subjects least efficient (with greatest activation). Thus, COMT appears to be a gene affecting prefrontal information processing by virtue of its effect on prefrontal dopamine signaling.
Furthermore, the demonstration in the present study of decreased dorsolateral prefrontal cortex efficiency during working memory activation in persons at greater genetic risk for schizophrenia might explain the greater risk for schizophrenia attributable to the COMT Val allele per se. In the context of a noisy and inefficient dorsolateral prefrontal cortex in individuals at greater genetic risk for schizophrenia (implicated by our fMRI data and accounted for by presumably genetic and environmental factors), inheritance of the COMT Val allele may further compound this abnormality in information processing in the dorsolateral prefrontal cortex, pushing these individuals closer to the state of abnormal prefrontal function that is associated with manifest schizophrenia. We believe this is a plausible mechanism by which the COMT Val allele represents a susceptibility allele for schizophrenia. If individuals at genetic risk for schizophrenia were not abnormal in terms of the efficiency of information processing in the dorsolateral prefrontal cortex, inheritance of the COMT Val allele would not further increase risk for this particular illness.
In conclusion, we found that unaffected siblings of schizophrenia patients had lower physiological efficacy of the dorsolateral prefrontal cortex during working memory, even without manifest working memory deficits. We replicated this finding in a second independent group. The observation that dorsolateral prefrontal cortex inefficiency during these conditions is related to genetic risk for schizophrenia may explain why inheritance of the COMT Val allele is associated with a slightly greater susceptibility to manifest schizophrenia (45). The COMT Val contributes to dorsolateral prefrontal cortex inefficiency during working memory activation presumably because of its effects on dopamine signaling and may interact with the effects of other genetic and environmental causes of physiological inefficacy of the dorsolateral prefrontal cortex. That the dorsolateral prefrontal cortex under our conditions was found in unaffected siblings diminishes concerns that earlier demonstrations of dorsolateral prefrontal cortex inefficiency in schizophrenia patients (27–29) arose from illness-associated confounds such as antipsychotic medication or the effects of chronic mental illness. Although formal estimates of heritability remain to be determined, we infer that fMRI measures of neuronal function of the dorsolateral prefrontal cortex likely represent a promising approach to identifying candidate intermediate phenotypes for the identification of susceptibility genes in schizophrenia. At a more basic level, abnormal information processing in the dorsolateral prefrontal cortex appears to be a component of biological susceptibility for schizophrenia.
![]() |
![]() |
Received Jan. 24, 2002; revisions received July 24 and Oct. 29, 2002; accepted Oct. 31, 2002. From the Clinical Brain Disorders Branch, NIMH, NIH. Address reprint requests to Dr. Callicott, Clinical Brain Disorders Branch, IRP, NIMH, NIH, Bldg. 10, Center Dr., Rm. 4D-20 MSC1389, Bethesda, MD 20982-1389; [email protected] (e-mail). Supported by the NIMH Intramural Research Program (a grant to the Clinical Brain Disorders Branch), the Stanley Foundation (a grant to Dr. Weinberger), and a Young Investigator Award from the National Alliance for Research in Schizophrenia and Depression (to Dr. Callicott). The authors thank the following for their help: Terry Goldberg, Richard Coppola, Karen Jones, Frederick Langheim, Daniel Podell, Karen Berman, Andreas Meyer-Lindenberg, and the staff of the Clinical Brain Disorders Branch Sibling Study and all participating families whose participation made this project possible.
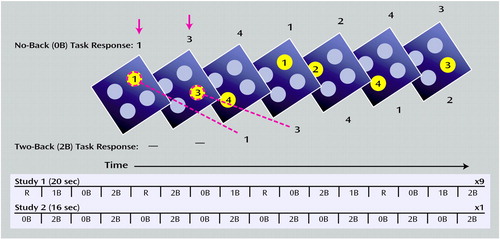
Figure 1. N-Back Working Memory Task and Experimental Designs of Two Studies Comparing Brain Activation in Unaffected Siblings of Patients With Schizophrenia and Healthy Comparison Subjectsa
aSubjects viewed one diamond at a time. During the 0-back task, subjects identified the number currently seen. During the 2-back task, subjects encoded the current stimulus while indicating the number seen two stimuli previously. At the bottom, the general outlines for fMRI data acquisition are presented for studies 1 and 2. In study 1, 20-second periods of rest (R) and 0-back, 1-back, and 2-back tasks were pseudorandomly presented in blocks consisting of eight tasks. Nine blocks were collected in total. In study 2, one block alternating between 0-back and 2-back tasks was collected.
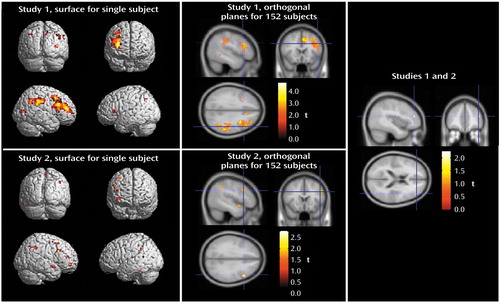
Figure 2. Abnormal Brain Activation Shown by Blood-Oxygen-Level-Dependent fMRI During N-Back Working Memory Task in Unaffected Siblings of Patients With Schizophrenia in Relation to Healthy Comparison Subjectsa
aSPM 99 analyses indicated areas in which siblings showed greater fMRI activation during the 2-back working memory task than the comparison subjects. The image at the upper left shows study 1 data rendered onto a canonical single-subject surface from SPM 99. The image at the upper center shows the same data presented in three orthogonal planes on a canonical average T1 brain from 152 subjects (SPM 99). The image at the lower left shows data from study 2 (replication study) rendered onto a canonical single-subject surface. The image at the lower center shows the same data presented in three orthogonal planes. The image at the right shows areas of significant activation shared by data from studies 1 and 2.
1. Gottesman II: Schizophrenia Genesis: Origins of Madness. San Francisco, WH Freeman, 1991Google Scholar
2. Risch N: Genetic linkage and complex diseases, with special reference to psychiatric disorders. Genet Epidemiol 1990; 7:3-16Crossref, Medline, Google Scholar
3. Faraone SV, Seidman LJ, Kremen WS, Pepple JR, Lyons MJ, Tsuang MT: Neuropsychological functioning among the nonpsychotic relatives of schizophrenic patients: a diagnostic efficiency analysis. J Abnorm Psychol 1995; 104:286-304Crossref, Medline, Google Scholar
4. Freedman R, Adler LE, Waldo MC, Pachtman E, Franks RD: Neurophysiological evidence for a defect in inhibitory pathways in schizophrenia: comparison of medicated and drug-free patients. Biol Psychiatry 1983; 18:537-551Medline, Google Scholar
5. Tsuang MT: Genotypes, phenotypes, and the brain: a search for connections in schizophrenia. Br J Psychiatry 1993; 163:299-307Crossref, Medline, Google Scholar
6. Weinberger DR, Egan MF, Bertolino A, Callicott JH, Mattay VS, Lipska BK, Berman KF, Goldberg TE: Prefrontal neurons and the genetics of schizophrenia: prefrontal neurons and the genetics of schizophrenia. Biol Psychiatry 2001; 50:825-844Crossref, Medline, Google Scholar
7. James JW: Frequency in relatives for an all-or-none trait. Ann Hum Genet 1971; 35:47-49Crossref, Medline, Google Scholar
8. Risch N: Linkage strategies for genetically complex traits, I: multilocus models. Am J Hum Genet 1990; 46:222-228Medline, Google Scholar
9. Egan MF, Weinberger DR: Neurobiology of schizophrenia. Curr Opin Neurobiol 1997; 7:701-707Crossref, Medline, Google Scholar
10. Braff DL, Callaway E, Naylor H: Very short-term memory dysfunction in schizophrenia: defective short-time constant information processing in schizophrenia. Arch Gen Psychiatry 1977; 34:25-30Crossref, Medline, Google Scholar
11. Cannon TD, Zorrilla LE, Shtasel D, Gur RE, Gur RC, Marco EJ, Moberg P, Price RA: Neuropsychological functioning in siblings discordant for schizophrenia and healthy volunteers. Arch Gen Psychiatry 1994; 51:651-661Crossref, Medline, Google Scholar
12. Goldberg TE, Torrey EF, Gold JM, Bigelow LB, Ragland RD, Taylor E, Weinberger DR: Genetic risk of neuropsychological impairment in schizophrenia: a study of monozygotic twins discordant and concordant for the disorder. Schizophr Res 1995; 17:77-84Crossref, Medline, Google Scholar
13. Park S, Holzman PS, Goldman-Rakic PS: Spatial working memory deficits in the relatives of schizophrenic patients. Arch Gen Psychiatry 1995; 52:821-828Crossref, Medline, Google Scholar
14. Arolt V, Lencer R, Nolte A, Muller-Myhsok B, Purman S, Schurmann M, Leutelt J, Pinnow M, Schwinger E: Eye-tracking dysfunction is a putative phenotypic susceptibility marker of schizophrenia and maps to a locus on chromosome 6p in families with multiple occurrence of the disease. Am J Med Genet 1996; 67:564-579Crossref, Medline, Google Scholar
15. Freedman R, Coon H, Myles-Worsley M, Orr-Urteger A, Olincy A, Davis A, Polymeropoulus M, Holik J, Hopkins J, Hoff M, Rosenthal J, Waldo MC, Reimherr F, Wender P, Yaw J, Young DA, Breese CR, Adams C, Patterson D, Adler LE, Kruglyak L, Leonard S, Byerley W: Linkage of a neurophysiological deficit in schizophrenia to a chromosome 15 locus. Proc Natl Acad Sci USA 1997; 94:587-592Crossref, Medline, Google Scholar
16. Egan MF, Goldberg TE, Gscheidle T, Weirich M, Bigelow LB, Weinberger DR: Relative risk of attention deficits in siblings of patients with schizophrenia. Am J Psychiatry 2000; 157:1309-1316Link, Google Scholar
17. Keefe RS, Roitman SE, Harvey PD, Blum CS, DuPre RL, Prieto DM, Davidson M, Davis KL: A pen-and-paper human analogue of a monkey prefrontal cortex activation task: spatial working memory in patients with schizophrenia. Schizophr Res 1995; 17:25-33Crossref, Medline, Google Scholar
18. Carter C, Robertson L, Nordahl T, Chaderjian M, Kraft L, O’Shora-Celaya L: Spatial working memory deficits and their relationship to negative symptoms in unmedicated schizophrenia patients. Biol Psychiatry 1996; 40:930-932Crossref, Medline, Google Scholar
19. Wexler BE, Stevens AA, Bowers AA, Sernyak MJ, Goldman-Rakic PS: Word and tone working memory deficits in schizophrenia. Arch Gen Psychiatry 1998; 55:1093-1096Crossref, Medline, Google Scholar
20. Weickert TW, Goldberg TE, Gold JM, Bigelow LB, Egan MF, Weinberger DR: Cognitive impairments in patients with schizophrenia displaying preserved and compromised intellect. Arch Gen Psychiatry 2000; 57:907-913Crossref, Medline, Google Scholar
21. Weinberger DR, Berman KF, Zec RF: Physiologic dysfunction of dorsolateral prefrontal cortex in schizophrenia, I: regional cerebral blood flow evidence. Arch Gen Psychiatry 1986; 43:114-124Crossref, Medline, Google Scholar
22. Catafau AM, Parellada E, Lomena FJ, Bernardo M, Pavia J, Ros D, Setoain J, Gonzalez-Monclus E: Prefrontal and temporal blood flow in schizophrenia: resting and activation technetium-99m-HMPAO SPECT patterns in young neuroleptic-naive patients with acute disease. J Nucl Med 1994; 35:935-941Medline, Google Scholar
23. Goldman-Rakic PS: Prefrontal cortical dysfunction in schizophrenia: the relevance of working memory, in Psychopathology and the Brain. Edited by Carroll BJ, Barnett JE. New York, Raven Press, 1991Google Scholar
24. Goldberg TE, Kelsoe JR, Weinberger DR, Pliskin NH, Kirwin PD, Berman KF: Performance of schizophrenic patients on putative neuropsychological tests of frontal lobe function. Int J Neurosci 1988; 42:51-58Crossref, Medline, Google Scholar
25. Cannon TD, Huttunen MO, Lonnqvist J, Tuulio-Henriksson A, Pirkola T, Glahn D, Finkelstein J, Hietanen M, Kaprio J, Koskenvuo M: The inheritance of neuropsychological dysfunction in twins discordant for schizophrenia. Am J Hum Genet 2000; 67:369-382Crossref, Medline, Google Scholar
26. Conklin HM, Curtis CE, Katsanis J, Iacono WG: Verbal working memory impairment in schizophrenia patients and their first-degree relatives: evidence from the Digit Span Task. Am J Psychiatry 2000; 157:275-277Link, Google Scholar
27. Manoach DS, Press DZ, Thangaraj V, Searl MM, Goff DC, Halpern E, Saper CB, Warach S: Schizophrenic subjects activate dorsolateral prefrontal cortex during a working memory task, as measured by fMRI. Biol Psychiatry 1999; 45:1128-1137Crossref, Medline, Google Scholar
28. Manoach DS, Gollub RL, Benson ES, Searl MM, Goff DC, Halpern E, Saper CB, Rauch SL: Schizophrenic subjects show aberrant fMRI activation of dorsolateral prefrontal cortex and basal ganglia during working memory performance. Biol Psychiatry 2000; 48:99-109Crossref, Medline, Google Scholar
29. Callicott JH, Bertolino A, Mattay VS, Langheim FJ, Duyn J, Coppola R, Goldberg TE, Weinberger DR: Physiological dysfunction of the dorsolateral prefrontal cortex in schizophrenia revisited. Cereb Cortex 2000; 10:1078-1092Crossref, Medline, Google Scholar
30. Ingvar DH, Franzen G: Distribution of cerebral activity in chronic schizophrenia. Lancet 1974; 2:1484-1486Crossref, Medline, Google Scholar
31. Frith CD, Friston KJ, Herold S, Silbersweig D, Fletcher P, Cahill C, Dolan RJ, Frackowiak RS, Liddle PF: Regional brain activity in chronic schizophrenic patients during the performance of a verbal fluency task. Br J Psychiatry 1995; 167:343-349Crossref, Medline, Google Scholar
32. Taylor SF: Cerebral blood flow activation and functional lesions in schizophrenia. Schizophr Res 1996; 19:129-140Crossref, Medline, Google Scholar
33. Carter CS, Perlstein W, Ganguli R, Brar J, Mintun M, Cohen JD: Functional hypofrontality and working memory dysfunction in schizophrenia. Am J Psychiatry 1998; 155:1285-1287Link, Google Scholar
34. Spence SA, Hirsch SR, Brooks DJ, Grasby PM: Prefrontal cortex activity in people with schizophrenia and control subjects: evidence from positron emission tomography for remission of “hypofrontality” with recovery from acute schizophrenia. Br J Psychiatry 1998; 172:316-323; correction, 172:543Crossref, Medline, Google Scholar
35. Callicott JH, Ramsey NF, Tallent K, Bertolino A, Knable MB, Coppola R, Goldberg T, van Gelderen P, Mattay VS, Frank JA, Moonen CT, Weinberger DR: Functional magnetic resonance imaging brain mapping in psychiatry: methodological issues illustrated in a study of working memory in schizophrenia. Neuropsychopharmacology 1998; 18:186-196Crossref, Medline, Google Scholar
36. Stevens AA, Goldman-Rakic PS, Gore JC, Fulbright RK, Wexler BE: Cortical dysfunction in schizophrenia during auditory word and tone working memory demonstrated by functional magnetic resonance imaging. Arch Gen Psychiatry 1998; 55:1097-1103Crossref, Medline, Google Scholar
37. Curtis VA, Bullmore ET, Morris RG, Brammer MJ, Williams SC, Simmons A, Sharma T, Murray RM, McGuire PK: Attenuated frontal activation in schizophrenia may be task dependent. Schizophr Res 1999; 37:35-44Crossref, Medline, Google Scholar
38. Honey GD, Bullmore ET, Soni W, Varatheesan M, Williams SC, Sharma T: Differences in frontal cortical activation by a working memory task after substitution of risperidone for typical antipsychotic drugs in patients with schizophrenia. Proc Natl Acad Sci USA 1999; 96:13432-13437Crossref, Medline, Google Scholar
39. Toone BK, Okocha CI, Sivakumar K, Syed GM: Changes in regional cerebral blood flow due to cognitive activation among patients with schizophrenia. Br J Psychiatry 2000; 177:222-228Crossref, Medline, Google Scholar
40. Spence SA, Liddle PF, Stefan MD, Hellewell JS, Sharma T, Friston KJ, Hirsch SR, Frith CD, Murray RM, Deakin JF, Grasby PM: Functional anatomy of verbal fluency in people with schizophrenia and those at genetic risk: focal dysfunction and distributed disconnectivity reappraised. Br J Psychiatry 2000; 176:52-60Crossref, Medline, Google Scholar
41. Barch D, Carter C, Braver T, Sabb F, MacDonald A, Noll D, Cohen J: Selective deficits in prefrontal cortex function in medication-naive patients with schizophrenia. Arch Gen Psychiatry 2001; 58:280-288Crossref, Medline, Google Scholar
42. Li T, Sham PC, Vallada H, Xie T, Tang X, Murray RM, Liu X, Collier DA: Preferential transmission of the high activity allele of COMT in schizophrenia. Psychiatr Genet 1996; 6:131-133Crossref, Medline, Google Scholar
43. Kunugi H, Vallada HP, Sham PC, Hoda F, Arranz MJ, Li T, Nanko S, Murray RM, McGuffin P, Owen M, Gill M, Collier DA: Catechol-O-methyltransferase polymorphisms and schizophrenia: a transmission disequilibrium study in multiply affected families. Psychiatr Genet 1997; 7:97-101Crossref, Medline, Google Scholar
44. Li T, Ball D, Zhao J, Murray RM, Liu X, Sham PC, Collier DA: Family-based linkage disequilibrium mapping using SNP marker haplotypes: application to a potential locus for schizophrenia at chromosome 22q11. Mol Psychiatry 2000; 5:77-84Crossref, Medline, Google Scholar
45. Egan MF, Goldberg TE, Kolachana BS, Callicott JH, Mazzanti CM, Straub RE, Goldman D, Weinberger DR: Effect of COMT Val 108/158 Met genotype on frontal lobe function and risk for schizophrenia. Proc Natl Acad Sci USA 2001; 98:6917-6922Crossref, Medline, Google Scholar
46. Callicott JH, Mattay VS, Bertolino A, Finn K, Jones K, Frank JA, Goldberg TE, Weinberger DR: Physiological characteristics of capacity constraints in working memory as revealed by functional MRI. Cereb Cortex 1999; 9:20-26Crossref, Medline, Google Scholar
47. First MB, Spitzer RL, Gibbon M, Williams JBW: Structured Clinical Interview for DSM-IV Axis I Disorders (SCID). Washington, DC, American Psychiatric Press, 1997Google Scholar
48. Weinberger DR, Mattay V, Callicott J, Kotrla K, Santha A, van Gelderen P, Duyn J, Moonen C, Frank J: fMRI applications in schizophrenia research. Neuroimage 1996; 4(3, part 3):S118-S126Google Scholar
49. Oldfield RC: The assessment and analysis of handedness: the Edinburgh Inventory. Neuropsychologia 1971; 9:97-113Crossref, Medline, Google Scholar
50. Hollingshead AB, Redlich FC: Social Class and Mental Illness: A Community Study. New York, John Wiley & Sons, 1958Google Scholar
51. Jastak S, Wilkinson GS: The Wide Range Achievement Test, Revised. Wilmington, Del, Jastak Associates, 1985Google Scholar
52. Bandettini PA, Wong EC, Hinks RS, Tikofsky RS, Hyde JS: Time course EPI of human brain function during task activation. Magn Reson Med 1992; 25:390-397Crossref, Medline, Google Scholar
53. Mattay VS, Frank JA, Santha AK, Pekar JJ, Duyn JH, McLaughlin AC, Weinberger DR: Whole-brain functional mapping with isotropic MR imaging. Radiology 1996; 201:399-404Crossref, Medline, Google Scholar
54. Yang Y, Glover GH, van Gelderen P, Mattay VS, Santha AK, Sexton RH, Ramsey NF, Moonen CT, Weinberger DR, Frank JA, Duyn JH: Fast 3D functional magnetic resonance imaging at 1.5 T with spiral acquisition. Magn Reson Med 1996; 36:620-626Crossref, Medline, Google Scholar
55. Ostuni JL, Santha AK, Mattay VS, Weinberger DR, Levin RL, Frank JA: Analysis of interpolation effects in the reslicing of functional MR images. J Comput Assist Tomogr 1997; 21:803-810Crossref, Medline, Google Scholar
56. Woods RP, Grafton ST, Watson JDG, Sicotte NL, Mazziotta JC: Automated image registration, I: general methods and intrasubject, intramodality validation. J Comput Assist Tomogr 1998; 22:139-152Crossref, Medline, Google Scholar
57. Evans AC, Marrett S, Neelin P, Collins L, Worsley K, Dai W, Milot S, Meyer E, Bub D: Anatomical mapping of functional activation in stereotactic coordinate space. Neuroimage 1992; 1:43-53Crossref, Medline, Google Scholar
58. Mazziotta JC, Toga AW, Evans A, Fox P, Lancaster J: A probabilistic atlas of the human brain: theory and rationale for its development. Neuroimage 1995; 2:89-101Crossref, Medline, Google Scholar
59. Friston KJ, Holmes AP, Worsley KJ, Poline JB, Frith CD, Frackowiak RSJ: Statistical parametric mapping in functional imaging: a general approach. Hum Brain Mapp 1995; 2:189-210Crossref, Google Scholar
60. Talairach J, Tournoux P: Co-Planar Stereotaxic Atlas of the Human Brain: Three-Dimensional Proportional System. New York, Thieme Medical, 1988Google Scholar
61. Smith EE, Jonides J: Neuroimaging analyses of human working memory. Proc Natl Acad Sci USA 1998; 95:12061-12068Crossref, Medline, Google Scholar
62. Perlstein WM, Carter CS, Noll DC, Cohen JD: Relation of prefrontal cortex dysfunction to working memory and symptoms in schizophrenia. Am J Psychiatry 2001; 158:1105-1113Link, Google Scholar
63. Bertolino A, Esposito G, Callicott JH, Mattay VS, Van Horn JD, Frank JA, Berman KF, Weinberger DR: Specific relationship between prefrontal neuronal N-acetylaspartate and activation of the working memory cortical network in schizophrenia. Am J Psychiatry 2000; 157:26-33Link, Google Scholar
64. Grady CL, Craik FI: Changes in memory processing with age. Curr Opin Neurobiol 2000; 10:224-231Crossref, Medline, Google Scholar
65. Rypma B, D’Esposito M: Isolating the neural mechanisms of age-related changes in human working memory. Nat Neurosci 2000; 3:509-515Crossref, Medline, Google Scholar
66. Freedman R, Leonard S, Gault JM, Hopkins J, Cloninger CR, Kaufmann CA, Tsuang MT, Faraone SV, Malaspina D, Svrakic DM, Sanders A, Gejman P: Linkage disequilibrium for schizophrenia at the chromosome 15q13-14 locus of the alpha7-nicotinic acetylcholine receptor subunit gene (CHRNA7). Am J Med Genet 2001; 105:20-22Crossref, Medline, Google Scholar
67. Mannisto PT, Kaakkola S: Catechol-O-methyltransferase (COMT): biochemistry, molecular biology, pharmacology, and clinical efficacy of the new selective COMT inhibitors. Pharmacol Rev 1999; 51:593-628Medline, Google Scholar