N-Methyl-d-Aspartic Acid Receptor Expression in the Dorsolateral Prefrontal Cortex of Elderly Patients With Schizophrenia
Abstract
OBJECTIVE: The N-methyl-d-aspartic acid (NMDA) class of glutamate receptors has received attention in the pathophysiology of schizophrenia because of the similarity between some schizophrenic symptoms and symptoms caused by NMDA antagonists. To determine if NMDA receptor abnormalities were present at the mRNA level, expression of NMDA receptor (NR) subunits NR1, NR2A, and NR2B was measured in specimens from the dorsolateral prefrontal cortex and the occipital cortex of elderly patients with schizophrenia and normal elderly subjects. METHOD: Postmortem specimens from antemortem assessed and diagnosed elderly patients with schizophrenia (N=26) were compared with those from a neuropathologically and neuropsychiatrically normal elderly comparison group (N=13) and from patients with Alzheimer’s disease (N=10). The mRNA expression of the NR1, NR2A, and NR2B subunits and of postsynaptic density 95 (PSD-95), a protein associated with postsynaptic NMDA receptors, was studied with quantitative real-time reverse transcriptase polymerase chain reaction. RESULTS: Expression of NR1 and NR2A but not NR2B subunits was higher in the dorsolateral prefrontal cortex and the occipital cortex of patients with schizophrenia than in the normal and Alzheimer’s disease groups. In contrast, NR1 expression was significantly lower in the Alzheimer’s disease group. Occipital cortex expression of PSD-95 was higher in the schizophrenic subjects and correlated strongly with the expression of NR2A and NR2B in both cortical regions and with expression of NR1 in the occipital cortex. These results were not influenced by neuroleptic exposure history, postmortem interval, or age of the subject. CONCLUSIONS: NMDA receptor subunits are abnormally expressed in elderly patients with schizophrenia. The disproportionate expression of the NR1 and NR2A subunits relative to NR2B expression may have implications for the pathophysiology of schizophrenia and the sensitivity of schizophrenic patients to glutamate and glutamatergic drugs.
Several neurochemical hypotheses have been proposed to explain the origin of schizophrenia, including abnormal dopamine, serotonin (5-HT), γ-aminobutyric acid (GABA), and/or glutamate neurotransmission in different regions of the brain (1–8). Abnormalities in the dorsolateral prefrontal cortex have figured prominently in many of these hypotheses, in part due to results of in vivo imaging and neuroanatomical studies (9–11), although there is evidence for structural, metabolic, and neurochemical abnormalities in many other brain regions, including the thalamus, the hippocampus, and the cingulate and entorhinal cortices (1, 12–19).
Strong evidence supporting an association between glutamatergic hypofunction and schizophrenia has come from pharmacological studies showing that N-methyl-d-aspartic acid (NMDA) receptor antagonists such as phencyclidine and ketamine can induce many of the psychotic signs and symptoms of schizophrenia in normal subjects, as well as exacerbate these signs and symptoms in subjects with schizophrenia (20–27). Observations of abnormalities in markers of glutamatergic neurotransmission in the hippocampus and the entorhinal, cingulate, orbital, and prefrontal cortices of patients with schizophrenia (3, 13, 28–43), the critical role of glutamatergic systems in learning and memory (44, 45), and the clear evidence for the neurotoxicity of glutamate (28, 46, 47) have given further credence to the potential involvement of the glutamatergic system in schizophrenia.
Glutamate receptors comprise four different receptor families: NMDA, kainate, α-amino-3-hydroxy-5-methylisoxazole-4-propionate (AMPA), and metabotropic receptors (48, 49). Because glutamate is the principal excitatory neurotransmitter in the brain, its receptors are ubiquitously but relatively discretely distributed throughout the neuraxis (50–53). Although there is evidence suggesting that kainate receptors are predominantly presynaptic and that AMPA and NMDA receptors are predominantly postsynaptic and often coexpressed, AMPA and NMDA receptors can also be localized to presynaptic sites (51, 54). Each receptor is assembled from multiple subunits that are encoded by different genes. The NMDA receptor is assembled from a combination of four–five subunits designated NR1 and NR2A–D. The NR1 subunit is often considered obligatory for functional NMDA receptor assemblies, but the remaining subunits can vary. Additional heterogeneity is conferred by eight alternative splice variants of the NR1 subunit that have different expression patterns within the human brain (55). The pattern of subunits assembled to form specific glutamate receptors varies from brain region to brain region (55). In the cerebral cortex and the hippocampus, NMDA receptors are assembled from NR1, NR2A, and NR2B subunits, and the NR2C and NR2D subunits are generally thought not to be expressed at appreciable levels (54, 56) (but see reference 38). Since the various combinations of NMDA receptor subunits confer different sensitivities to various endogenous and exogenous glutamatergic ligands and since NMDA receptors can be localized pre- and postsynaptically (57–59), the release of glutamate from any given neuron or a pharmacologic glutamate ligand can have a wide range of effects in different brain regions and on glutamatergic function and activity.
At a postsynaptic level, the clustering, assemblage, and anchoring of NMDA receptors is governed in part by a protein family known as postsynaptic density 95/synapse-associated protein 90 (PSD-95/SAP-90) that appears also to play an important role in the binding and assemblage of signal transduction complexes (60–65). PSD-95 is exclusively localized with postsynaptic NMDA receptor-related densities (62, 66), with significantly greater association with the NR2A and NR2B subunits than with the NR1 subunit, although it does co-localize and associate with some splice variants of the NR1 subunit (60, 62). PSD-95 also plays an important role in signal transduction, nitric oxide neurotoxicity linked to NMDA receptor activation (67, 68), and NMDA-induced long-term potentiation (69). Because of these characteristics, PSD-95 is important in providing a functional scaffold for postsynaptic NMDA receptors and in mediating intracellular NMDA receptor functions.
The study described here sought to determine whether the expression of genes encoding for NR1, NR2A, and NR2B subunits of the NMDA receptor and their postsynaptic anchoring protein PSD-95 are specifically altered in the dorsolateral prefrontal cortex of patients with schizophrenia. The dorsolateral prefrontal cortex was studied in postmortem brains of elderly schizophrenic subjects who had been antemortem diagnosed with DSM-IV criteria (70–72), had no other neuropsychiatric disease, had died of natural nonviolent causes without coma, had no evidence of significant neuropathology (73), and had clear documentation of neuroleptic drug exposure during the months and weeks before death. Specimens of the dorsolateral prefrontal cortex from these subjects were compared to identically treated and dissected specimens from normal elderly subjects who were found by chart review and caregiver interviews to have no neuropsychiatric or neurological diseases and who had no significant neuropathology. Specificity to schizophrenia was tested by the inclusion of specimens from patients with Alzheimer’s disease. To determine the specificity of findings to the dorsolateral prefrontal cortex, specimens from the primary occipital cortex (Brodmann’s area 17) from the same subjects were dissected and assessed for NMDA receptor and PSD-95 transcript mRNA abundance by using identical procedures.
Method
Human Postmortem Tissue
Frozen postmortem brain samples from subjects diagnosed with DSM-IV schizophrenia (N=26), normal elderly comparison subjects (N=13), and subjects with Alzheimer’s disease (N=10) were obtained from the Brain Bank, Department of Psychiatry, Mount Sinai/Bronx Veterans Administration Medical Center. The sex distribution and mean age, postmortem interval, and tissue pH of the cohorts are shown in Table 1. All schizophrenic subjects had been hospitalized for the long term at Pilgrim Psychiatric Center (New York). Complete medical charts were available for all subjects, and 16 of the 26 schizophrenic subjects had been prospectively diagnosed and neuropsychiatrically assessed by a team of research clinicians (70–72). Patients who died before the antemortem assessment by the research team were diagnosed by the same team of research clinicians who conducted diagnostic reviews of all medical charts (73). The reliability of these postmortem diagnostic procedures was confirmed by assessing an independent group of 35 subjects from the same institution by using structured interviews and blindly by using chart review. Interrater/interassessment reliability was 0.86. All assessment and postmortem evaluations and procedures were approved by the institutional review boards of the Pilgrim Psychiatric Center, the Mount Sinai School of Medicine, and the Bronx VA Medical Center. All patients had thorough neuropathologic characterization to rule out associated neurologic complications such as Alzheimer’s disease and multi-infarct dementia (73). Normal comparison subjects had no history of any psychiatric or neurologic disorders and no discernible neuropathologic lesions. Nine of the schizophrenic subjects had not received neuroleptic medications for at least 6 weeks before death (range=0–124 weeks). For comparison purposes and to enable assessment of specificity of the findings to schizophrenia, brain tissue from 10 subjects with a diagnosis of definite Alzheimer’s disease (according to criteria of the Consortium to Establish a Registry for Alzheimer’s Disease) (74) was also studied. The overall characteristics and diagnostic procedures for these Alzheimer’s disease subjects have been described extensively (75, 76).
Each brain was divided midsagittally at the time of extraction. The left half was sectioned in 6–8-mm coronal slabs, immediately snap-frozen in liquid nitrogen-cooled isopentane, and stored at –80°C. Gray matter from the frozen dorsolateral prefrontal cortex (77, 78) (Brodmann’s area 46) and occipital cortex (Brodmann’s area 17) was dissected from coronal sections of frozen brain (–80°C). Brodmann’s area 17 was identified as the area containing the band of Gennari in the coronal section from approximately 2 cm rostral to the occipital pole. The dissected tissues were pulverized at –190°C into a fine powder, aliquoted into individual Eppendorf tubes, and stored at –80°C until use.
Quantitation of NMDA Receptor Expression
RNA isolation
Total RNA was isolated from 50 mg of tissue with the guanidinium isothiocyanate method (79) by using the ToTALLY RNA kit (Ambion, Austin, Tex.) according to the manufacturer’s protocol. To remove genomic DNA contamination, isolated RNA samples were then treated with 40 units of DNase I (Ambion) in the presence of 120 units of RNaseOUT (GibcoBRL, Invitrogen, Carlsbad, Calif.) for 1 hour at 37°C. The yield of total RNA determined by absorbance at 260 nM ranged from 15 to 30 μg per 50 mg of brain tissue. The 260/280 nM ratios of the samples were >2.1. The yield and quality of total RNA was also analyzed by using agarose gel electrophoresis.
Reverse transcriptase reaction
Total RNA (∼2 μg) was used in 20 μl of reverse transcriptase reaction to synthesize cDNA, by using a ThermoScript RT-PCR System kit (GibcoBRL) and random hexamers as primers. The cDNA was diluted 50 times with water, and 5 μl of the diluted cDNA was amplified in 25 μl of polymerase chain reaction (PCR) mix.
Primer and molecular beacon design
Real-time reverse transcriptase polymerase chain reaction (RT-PCR) was used for quantitation of NMDA receptor and PSD-95 expression. PCR primers were designed by using Vector NTI software (InforMax, North Bethesda, Md.). NR1 primers were designed to ignore distinctions between known splicing variants of this NMDA receptor subunit. Molecular beacons were used as fluorogenic probes in the real-time PCR. Molecular beacons are hairpin-shaped molecules with an internally quenched fluorophore whose fluorescence is restored when they bind to their target nucleic acids (80–83). The molecular beacons were designed by using a DNA folding program (available at http://www.ibc.wustl.edu/∼zuker) to estimate the stability of the hairpin stem. The molecular beacons used in the experiments contained probe sequences that were 22–25 nucleotides long and arm sequences that were six nucleotides long. The melting temperature of the hairpin stems and probe sequences was 64–66°C. Fluorescein (FAM) or tetrachloro 6-carboxyfluorescein (TET) fluorophores were covalently linked to the 5′ end of the molecular beacons, and the quencher 4-(4′-dimethylaminophenylazo) benzoic acid (DABCYL) was covalently linked to the 3′ end. The primers and molecular beacons were synthesized either commercially (IDT, Coralville, Iowa) or by one of us (S.A.E.M.). The primer pair and molecular beacon sequences that were used to detect each of the mRNAs are shown in Table 2.
Real-time PCR
Real-time PCR analysis was performed by using an ABI Prism 7700 Sequence Detector (Applied Biosystems, Foster City, Calif.). Each 25 μl PCR reaction contained 5 μl of the relevant cDNA, 200 nM of molecular beacon, 500 nM of each primer, 1 unit of AmpliTaq Gold DNA polymerase (Applied Biosystems), 250 μM of each deoxy-nucleotide triphosphate (dNTP), 4 mM MgCl2, 50 mM KCl, and 10 mM Tris-HCl (pH 8.3). The thermal cycling program consisted of 10 minutes at 95°C to activate the polymerase, followed by 10 cycles of 15 seconds at 95°C and 45 seconds at 70–61°C (touch-down PCR, annealing temperature was decreased 1°C after each cycle). This touch-down step was followed by 35 cycles of 15 seconds at 95°C and 1 minute at 60°C. Fluorescence was monitored during the 60°C annealing-extension steps. The reactions were quantitated by selecting the amplification cycle when the PCR product of interest was first detected (threshold cycle [Ct]).
To determine sensitivity of the assays, the amplification of each mRNA in serial dilutions of cDNA derived from pooling of human cortical specimens from 10 randomly selected subjects (pooled sample) was measured. Figure 1 shows the amplification of NR1 mRNA in 10-fold dilutions of pooled cDNA and the threshold cycle values of these amplifications plotted against the log of the relative initial amount of cDNA. In assays that use PCR to amplify a target sequence exponentially, there is an inverse linear relationship between the threshold cycle and the logarithm of the number of target molecules that were present initially (84). Theoretically, the slope of this linear curve is expected to be –3.32. In the results shown on Figure 1, a linear relationship between threshold cycle and the initial amount of NR1 mRNA was demonstrated for four orders of magnitude. The slope of the curve was –3.23, which was very close to theoretically expected value. These data show the broad dynamic range of the NR1 mRNA quantitation. Similar results were obtained for each amplification assay performed in this study.
To account for different degrees of RNA degradation and other technical artifacts, relative quantitations of the expression levels of NR1, NR2A, NR2B and PSD-95 genes were performed as described in User Bulletin #2 for the ABI PRISM 7700 Sequence Detection System: Relative Quantitation of Gene Expression; Comparative Ct Method: Separate Tubes, product #4303859 (Applied Biosystems). The expression level of each gene of interest was normalized to the expression level of the endogenous reference (β-actin) in each sample. This relative value was further normalized to the relative expression of the same gene in the pooled sample (see the preceding paragraph). Pooled cDNAs were run in every plate simultaneously with experimental samples. To avoid competition, only one mRNA was amplified in each PCR (monoplex). All samples were run in triplicate.
To measure the level of contamination with chromosomal DNA, all RNA samples that were not treated with reverse transcriptase were subjected to PCR by using NR1 or NR2A primers. The products of the PCRs were analyzed on EtBr-stained agarose gels. In contrast to the respective cDNA templates, the RNA samples showed no PCR products. Random RNA samples (N=10) were also subjected to real-time PCR by using β-actin primers and a molecular beacon. The RNA samples showed at least 4.5 orders of magnitude fewer initial template molecules than the respective cDNAs templates (the difference in threshold cycle between RNAs and cDNA in each sample was at least 15 cycles), demonstrating negligible amounts of genomic DNA contamination (data not shown).
NMDA Receptor Expression in Neuroleptic-Treated Rats
To assess the effects of neuroleptic exposure on NMDA receptor mRNA, groups of six male Sprague-Dawley rats (6–8 months of age) received daily subcutaneous injections of haloperidol (2 mg/kg) or saline vehicle for 21 days. The specific haloperidol dosing parameters were selected because previous studies (85) have shown them to be effective in regulating dopamine receptor mRNA expression in the rat brain. The rats were sacrificed by decapitation 24 hours after the last injection, and their brains were rapidly removed. Cortices were dissected and immediately frozen on dry ice. NMDA receptor expression was assessed by using the procedures described earlier except that primers and molecular beacons specific to rat NMDA receptor subtypes were used (Table 2).
Statistical Analysis
Analysis of variance (ANOVA) and covariance (ANCOVA) followed by Newman-Keuls tests and t tests were used to analyze the results of these studies. ANOVA was used for analyses of data from the entire cohort. ANCOVA was used for analyses of data from the entire cohort, with age of the subject at the time of death as a covariate. T tests were used to compare differences between groups when the groups had been matched for age at the time of death. Because each brain region and each NMDA receptor subunit was measured independently with different probes and in different experiments, differences between groups for the expression of each gene were assessed with independent tests of significance. Pearson product moment correlations were used to assess the relationship between continuously distributed variables. Statistical analyses were performed with Statistica for Windows (release 5.5, Statsoft Inc., Tulsa, Okla.) or SPSS for Windows (version 10, SPSS Inc., Chicago).
Results
The relative abundance of NMDA receptor subunit expression in the dorsolateral prefrontal cortex and the occipital cortex for each group is shown in Figure 2. NR1 subunit expression in the dorsolateral prefrontal cortex was significantly higher in the schizophrenia group and significantly lower in the Alzheimer’s disease group, compared with the normal elderly group (F=12.75, df=2, 46, p=0.00004) (Newman-Keuls tests: schizophrenia versus normal elderly, p=0.009; Alzheimer’s disease versus normal elderly, p=0.05; Alzheimer’s disease versus schizophrenia, p=0.0002). Higher levels of NR1 subunit expression were evident in the occipital cortex of the schizophrenia subjects than in the normal subjects, but not in the Alzheimer’s disease subjects (F=6.79, df=2, 46, p=0.003) (Newman-Keuls tests: schizophrenia versus normal elderly, p=0.02; Alzheimer’s disease versus normal elderly, p=0.94). Expression of NR2A in the dorsolateral prefrontal cortex was not significantly different in either the schizophrenic or Alzheimer’s disease subjects relative to normal elderly subjects (p>0.14, Newman-Keuls). However, the level of NR2A expression in the occipital cortex of the schizophrenic subjects was significantly higher than that of the normal elderly subjects (F=5.5, df=2, 46, p=0.007; p=0.04, Newman-Keuls). The lower level of NR2A expression in the occipital cortex of the Alzheimer’s disease group, relative to the normal comparison group, did not reach statistical significance (p=0.40, Newman-Keuls). The expression of the NR2B subunit was not significantly altered in any group in either of the brain regions examined (F<0.9, df=2, 46, p>0.4). The expression of the mRNA for PSD-95 was also significantly higher in the schizophrenia subjects than in the normal elderly subjects (Figure 2). The schizophrenia-related difference in the expression of PSD-95 was most evident in the occipital cortex (F=4.31, df=2, 46, p=0.02; schizophrenia versus normal elderly, p=0.04, Newman-Keuls) but did not reach statistical significance in the dorsolateral prefrontal cortex (p=0.31, Newman-Keuls). PSD-95 gene expression was unchanged in the brains of the Alzheimer’s disease cohort.
The schizophrenic cohort was significantly younger than the normal elderly group (Table 1), and age at death correlated significantly with the expression of the NR1 and NR2A subunits of the NMDA receptor (r<−0.31, df=47, p<0.04) when the entire cohort was considered. The expression of the NMDA receptor subunits and PSD-95 mRNA did not correlate significantly with age within the schizophrenia cohort despite a relatively broad age range of 52–97 years. Two approaches were taken to determine whether age at death affected the differences between groups. First, ANCOVAs, with age as a covariate, were done. The inclusion of age as a covariate did not alter the pattern or the statistical significance of the results in any way (e.g., the ANCOVA comparing NR1 expression in the dorsolateral prefrontal cortex was significant [F=9.6, df=2, 45, p=0.0003]). Second, the schizophrenic and normal elderly groups were subgrouped into two groups of 10 subjects each, matched for age to within 1 year. The differences in the expression of the NMDA receptor subunits and PSD-95 were reassessed by using t tests. As for the ANCOVA, the same significant group differences (t<−2.2, df=18, p<0.04) found when analyzing the entire cohort were observed when comparing the age-matched schizophrenic and normal elderly subjects.
Table 3 shows the correlation of expression of the different NMDA receptor subunit mRNAs with each other and with PSD-95 in each brain region for the entire cohort. Nearly identical results were obtained when separate correlational analysis was performed for the schizophrenia subjects, the Alzheimer’s disease subjects, and the normal elderly subjects. Similarly, these correlations were not unduly influenced by differences in the age of the subjects, since the results were nearly identical when the contribution of age was factored out by using partial correlation analyses. PSD-95 gene expression correlated best with the expression of NR2A and NR2B in the dorsolateral prefrontal cortex and with NR1 gene expression in the occipital cortex but not with NR1 gene expression in the dorsolateral prefrontal cortex. Comparison of the correlations of PSD-95 with NR1, NR2A, and NR2B expression in the dorsolateral prefrontal cortex versus the occipital cortex (using Fisher’s r-to-z transformation [86]) showed that the correlations of PSD-95 with NR1 and NR2A were significantly stronger in the occipital cortex than in the dorsolateral prefrontal cortex (NR1: t=5.5, df=47, p<0.001; NR2A: t=4.1, df=47, p<0.001).
All of the schizophrenic subjects had been exposed to neuroleptics for decades. As mentioned previously, the history of neuroleptic exposure for each subject was assessed in detail by examining his or her medical chart. Of the 26 schizophrenic subjects, 13 had been exposed to neuroleptics to within 1 week of death, while neuroleptic medications had been discontinued for the remaining 13 subjects from 1 week before death to as long as 124 weeks before death. The neuroleptic-free interval did not correlate with the expression of any of the genes studied (r=–0.16 to 0.11, df=24, p>0.42). To further assess the possible influence of acute neuroleptic exposure on NMDA and PSD-95 gene expression, the schizophrenic group was subdivided into those who had been exposed to neuroleptics to within 6 weeks of death (N=16) and those who had been neuroleptic free more than 6 weeks (N=9) (data missing for one subject). Comparison of NR1, NR2A, NR2B, and PSD-95 gene expression in the dorsolateral prefrontal cortex and occipital cortex of these two groups did not reveal any significant differences (t tests, df=23, p>0.19, data not shown). As a further test of the potential influence of neuroleptic exposure on the expression of the genes of interest, NR1, NR2A, and NR2B gene expression was compared in the cortices of rats that had been treated with a daily 2-mg/kg dose of haloperidol for 3 weeks. No significant differences in NR1, NR2A, and NR2B gene expression were detected in the cortices of rats treated with haloperidol versus saline-treated rats (t<1.4, df=14, p>0.19).
Discussion
The results of this series of studies have shown that the expression of genes encoding for the predominant cortically expressed subunits of the NMDA receptor is abnormal in the dorsolateral prefrontal cortex and occipital cortex of schizophrenic subjects hospitalized for the long term who died of natural causes in old age. The expression of the NR1 subunit was consistently higher in both regions of the cortex in the schizophrenic subjects than in normal elderly comparison subjects, and the expression of the NR2A subunit was nominally higher in the dorsolateral prefrontal cortex and significantly higher in the occipital cortex in the schizophrenic subjects. The expression of the NR2B subunit was not significantly altered in either region in the schizophrenic subjects. In the schizophrenic subjects, the higher level of NMDA receptor subunit gene expression was accompanied by comparable higher levels of cortical-region-specific expression of PSD-95, which is associated with postsynaptic NDMA receptor specializations. This dysregulation in NMDA receptor subunit expression was specific to the schizophrenic subjects, insofar as identically treated and studied specimens from Alzheimer’s disease patients showed lower than normal levels of NR1 expression, as expected from previous studies (87, 88). Replication of the finding of lower levels of NR1 expression in Alzheimer’s disease confirms the validity of the methods used in this study and adds to the reliability of the findings in the schizophrenic tissues.
The altered expression pattern of the NMDA receptor subunits is unlikely to have been a result of the younger age of the schizophrenia group, because group differences persisted in analyses that included age as a covariate and were evident even when subgroups of schizophrenics and normal elderly subjects were matched closely for age. In addition, age did not correlate with the expression pattern of any of the genes in the schizophrenia cohort, despite those subjects’ relatively broad age range. It is possible that the observed differences between the normal elderly subjects and schizophrenic subjects resulted not only from schizophrenia but from an interaction between schizophrenia and the age of the subjects. Only replication of the study in a younger cohort can address this question. Similarly, it is noteworthy that the schizophrenic subjects in this study were chronically and severely ill and had required hospitalization for most of their lives. Whether the observed changes in NMDA receptor subunit and PSD-95 gene expression will generalize to less severely affected subjects is another question that must await replication of the study in a less severely affected cohort.
The possibility that the observed differences in NMDA and PSD-95 gene expression were influenced by exposure to neuroleptics cannot be excluded, but it is unlikely that the higher levels of NMDA receptor subunit gene expression were due to acute neuroleptic effects. Gene expression did not correlate with the amount of time subjects had been medication free before death; it was not differentially affected when the schizophrenia group was stratified into subgroups who had taken neuroleptics until the time of death versus those who had been neuroleptic free for 6–124 weeks; and it was not observed in the cortices of rats treated subchorionically with haloperidol for 3 weeks. That the observed NMDA receptor subunit gene expression was unlikely to have been directly influenced by neuroleptic exposure is supported further by other studies that have failed to find increases in cortical NMDA receptor gene expression after neuroleptic treatment (89–91).
The complexity of the glutamate system and its receptors is paralleled by the complexity of findings with respect to the expression of glutamate receptors in the brain in schizophrenia. Different studies have reported different findings in different regions of the brains of schizophrenic subjects. One study, which used in situ hybridization techniques and specimens from the same collection used here, reported higher levels of NR1 and NR2A expression in the prefrontal cortex of schizophrenic subjects (37), substantiating the results reported here with different detection techniques and different molecular probes. Other studies have reported higher levels of glutamate receptor binding in the orbital frontal cortex and the superior temporal gyrus that are in general agreement with the current findings (34, 35, 41). Results from another in situ hybridization study found that while the overall abundance of NMDA receptor subunits did not differ in the frontal cortices of schizophrenic subjects relative to comparison subjects, there was a shift toward increased abundance of the NR2 subunit class, especially NR2D subunits (38). Recently, Gao et al. (42) reported lower NR1 expression and higher NR2B expression in several subregions of the hippocampus of schizophrenic subjects, whereas the expression of the NR2A subunit was unchanged in the same regions. These and other studies (59) all support the conclusion that while the expression of glutamate receptors may be complex, it is nevertheless significantly affected in schizophrenia, and the expression of different subunits of NMDA receptors is significantly altered in different brain regions.
The observation of higher levels of NMDA receptor NR1 and NR2A subunit gene expression associated with schizophrenia raises the question of the functional consequences of this apparent change and its relationship to glutamatergic dysfunction hypotheses of schizophrenia. Knowledge of a possible disequilibrium in subunit expression does not directly provide an understanding of the attendant functional consequences, but the literature suggests that functional consequences are a likely result of altered NMDA gene expression (59). For example, receptors assembled in vitro from the NR1 subunit alone bind glycine antagonists, but the assembly of both NR1 and NR2A subunits is required for binding to glutamate antagonists and channel-blocking agents (92). Similarly, the channel properties and antagonist affinities of NMDA receptors assembled from combinations of NR1 and NR2A are different from receptors assembled from the NR1 and NR2B subunits (57, 58). The subunit composition of NMDA receptors can also significantly influence their susceptibility to neurotoxicity and to cell death. Cell lines transfected with NR1/NR2A subunits are more susceptible to cell death than those transfected with combinations of NR1/NR2B, which are more susceptible than cells transfected with NR1/NR2C subunits (47).
The potential functional, and perhaps deleterious, consequences of higher levels of NR1 and NR2A gene expression in schizophrenia are further suggested by the clear evidence for increased PSD-95 gene expression and the correlation of PSD-95 gene expression with NMDA receptor subunit expression. PSD-95 was significantly overexpressed in the occipital cortex, and its expression correlated most strongly with the expression of NMDA receptor subunit mRNA in this region, although correlations between PSD-95 mRNA and NR2A and NR2B mRNA in the dorsolateral prefrontal cortex were also significant and relatively high. Given the importance of the C-terminal domains of NR2A and NR2B to receptor function and to interaction with PSD-95 (93, 94), it is likely that the NMDA receptors formed by the higher levels of NR1 and NR2A subunits were appropriately clustered and anchored with at least some functional integrity. The coexistence of nitric oxide immunoreactivity and NMDA receptors on cortical spiny neurons (63, 68) and PSD-95 mediation of nitric oxide neurotoxicity induced by NMDA receptor activation (67) raise the possibility that higher than normal levels of NMDA receptor subunit expression have detrimental consequences.
The relationship between NR1, NR2A, and NR2B subunits and PSD-95 in the dorsolateral prefrontal cortex was different than that in the occipital cortex. PSD-95 expression correlated exceptionally strongly with NR1, NR2A, and NR2B expression in the occipital cortex, but it did not correlate significantly with NR1 subunit expression in the dorsolateral prefrontal cortex despite significantly higher levels of NR1 expression in both brain regions of schizophrenic subjects. Furthermore, PSD-95 correlated less strongly with NR2A and NR2B expression in the dorsolateral prefrontal cortex than in the occipital cortex. These results suggest that NMDA receptors are expressed predominantly at postsynaptic sites in the occipital cortex but that their distribution or composition may be different in the dorsolateral prefrontal cortex. These results also raise the possibility of local regulation of glutamatergic neurotransmission and suggest that different components of the glutamatergic systems may be affected differentially in different brain regions.
The divergent correlations between the NMDA receptor subunit and PSD-95 expression in the dorsolateral prefrontal cortex and occipital cortex could imply that the pre- and postsynaptic distribution of NMDA receptors is different in the two regions. Thus, if NMDA receptors were distributed both pre- and postsynaptically in the dorsolateral prefrontal cortex, but predominantly postsynaptically in the occipital cortex, then one would expect the correlations between the NMDA receptor subunits and PSD-95 to be significantly higher in the occipital cortex than in the dorsolateral prefrontal cortex. The lack of significantly higher levels of PSD-95 expression in the dorsolateral prefrontal cortex of the schizophrenic subjects would then suggest that the observed overexpression of the NR1 subunit in that region is likely presynaptic in origin. An alteration in the balance of pre- and postsynaptic NMDA receptors in the dorsolateral prefrontal cortex of schizophrenic subjects could have broad implications, not only with respect to glutamatergic function but also relative to the responsivity to glutamatergic agonists and antagonists.
A parsimonious, yet perhaps simplistic overall interpretation of the results of this study is that some NMDA receptors are more abundant in the dorsolateral prefrontal cortex and occipital cortex of schizophrenic subjects than in those regions in comparison subjects. This interpretation is concordant with a hypoglutamatergic state hypothesis of schizophrenia, especially given the strong possibility that the increased expression of at least some of these receptor subunits is at postsynaptic sites. A traditional pharmacological interpretation would suggest that lower levels of glutamatergic activity would lead to higher levels of expression of postsynaptic glutamate receptors. In fact, animal studies have shown that NMDA receptor antagonism with phencylclidine can increase the expression of NR1 mRNA (95). At first glance, however, this interpretation of the results is at odds with pharmacological studies of schizophrenia with NMDA receptor antagonists. Studies of the psychotomimetic effects of uncompetitive NMDA receptor antagonists have been instrumental in the development of hypotheses that posit a hypofunctional postsynaptic glutamatergic system in schizophrenia. Some recent evidence suggests that the interpretation of the results of studies with uncompetitive NMDA antagonists may be more complex and that the symptoms induced by ketamine could be a result of increased glutamate release and/or increased activation of postsynaptic glutamate receptors (24). Because of the presynaptic localization of some NMDA receptors (51, 54), a hyperglutamatergic state and increased glutamate release could result from ketamine administration and its interaction with presynaptic NMDA receptor elements (54, 96). The increased glutamate release could then act on postsynaptic glutamatergic receptors (e.g., AMPA) and provoke the psychotomimetic symptoms observed with glutamate receptor antagonists. If this interpretation of overexpressed NMDA receptors at presynaptic sites in the dorsolateral prefrontal cortex of schizophrenic patients is correct, then it would be reasonable to assume that NMDA antagonists would exacerbate the symptoms of schizophrenia. Thus, although the results of the current study and those cited earlier support the view that cortical glutamatergic systems are significantly affected and abnormal in schizophrenia, they highlight the need for further and more detailed studies to elucidate the precise nature of the glutamatergic abnormality.
![]() |
![]() |
![]() |
Received Nov. 16, 2000; revision received March 20, 2001; accepted March 27, 2001. From the Department of Psychiatry, Mount Sinai School of Medicine and Bronx Veterans Affairs Medical Center; and the Department of Molecular Genetics, Public Health Research Institute, New York. Address reprint requests to Dr. Haroutunian, Psychiatry Research (3F-02), Bronx VA Medical Center, 130 West Kingsbridge Rd., Bronx, NY 10468; [email protected] (e-mail). Supported by the Schizophrenia Brain Bank, Merit Review and Mental Illness Research, Education, and Clinical Center awards from the Department of Veterans Affairs to Dr. Haroutunian, NIMH grant MH-45212 to Dr. Davis, and grant HL-43521 from the National Heart, Lung, and Blood Institute to Dr. Kramer.
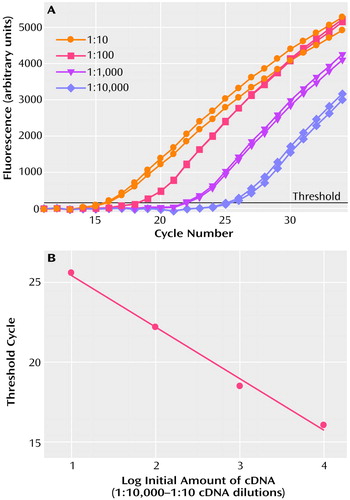
Figure 1. Dynamic Range of the mRNA Quantitation Assay for NMDA Receptor 1 (NR1)a
aPanel A: Amplification of NR1 mRNA in 10-fold dilutions of pooled cDNA. Panel B: Threshold values of amplifications of NR1 mRNA plotted against the log of the relative initial amount of cDNA. Similar results were obtained for the amplification assays performed for NR 2A, NR2B, PSD-95 and β-actin.
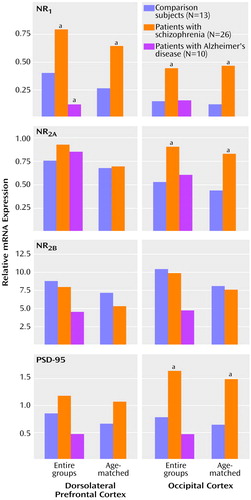
Figure 2. Relative Gene Expression of N-Methyl- d-Aspartic Acid (NMDA) Receptor Subunits NR1, NR2A, and NR 2B and of Postsynaptic Density 95 (PSD-95) in the Dorsolateral Prefrontal Cortex and the Occipital Cortex of Normal Comparison Subjects, Patients With Schizophrenia, and Patients With Alzheimer’s Disease
aSignificantly different from comparison group (p<0.05). Newman-Keuls tests were used for comparisons of entire groups. T tests (df=18) were used for comparisons of age-matched groups (10 patients with schizophrenia and 10 normal comparison subjects).
1. Benes FM: Emerging principles of altered neural circuitry in schizophrenia. Brain Res Brain Res Rev 2000; 31:251-269Crossref, Medline, Google Scholar
2. Tamminga CA: Glutamatergic aspects of schizophrenia. Br J Psychiatry Suppl 1999; 37:12-15Medline, Google Scholar
3. Carlsson A, Hansson LO, Waters N, Carlsson ML: A glutamatergic deficiency model of schizophrenia. Br J Psychiatry Suppl 1999; 37:2-6Medline, Google Scholar
4. Aghajanian GK, Marek GJ: Serotonin model of schizophrenia: emerging role of glutamate mechanisms. Brain Res Brain Res Rev 2000; 31:302-312Crossref, Medline, Google Scholar
5. Roth BL, Meltzer HY: The role of serotonin in schizophrenia, in Psychopharmacology: The Fourth Generation of Progress. Edited by Bloom FE, Kupfer DJ. New York, Raven Press, 1995, pp 1215-1227Google Scholar
6. Kahn RS, Davis KL: New developments in dopamine and schizophrenia. Ibid, pp 1193-1203Google Scholar
7. Chan Palay V, Lang W, Allen YS, Haesler U, Polak JM: Cortical neurons immunoreactive with antisera against neuropeptide Y are altered in Alzheimer’s-type dementia. J Comp Neurol 1985; 238:390-400Crossref, Medline, Google Scholar
8. Tamminga CA: Schizophrenia and glutamatergic transmission. Crit Rev Neurobiol 1998; 12:21-36Crossref, Medline, Google Scholar
9. Bunney WE, Bunney BG: Evidence for a compromised dorsolateral prefrontal cortical parallel circuit in schizophrenia. Brain Res Brain Res Rev 2000; 31:138-146Crossref, Medline, Google Scholar
10. Berman KF, Illowsky BP, Weinberger DR: Physiological dysfunction of dorsolateral prefrontal cortex in schizophrenia, IV: further evidence for regional and behavioral specificity. Arch Gen Psychiatry 1988; 45:616-622Crossref, Medline, Google Scholar
11. Casanova MF, Goldberg TE, Suddath RL, Daniel DG, Rawlings R, Lloyd DG, Loats HL, Kleinman JE, Weinberger DR: Quantitative shape analysis of the temporal and prefrontal lobes of schizophrenic patients: a magnetic resonance image study. J Neuropsychiatry Clin Neurosci 1990; 2:363-372Crossref, Medline, Google Scholar
12. Haroutunian V, Davis KL: Neuropathology of schizophrenia, in Current Issues in the Psychopharmacology of Schizophrenia. Edited by Breier A, Tran PV, Herrera J, Bymaster F, Tollefson GD. Baltimore, Williams & Wilkins, 2000, pp 57-70Google Scholar
13. Harrison PJ: The neuropathology of schizophrenia: a critical review of the data and their interpretation. Brain 1999; 122:593-624Crossref, Medline, Google Scholar
14. Hazlett EA, Buchsbaum MS, Byne W, Wei T-C, Spiegel-Cohen J, Geneve C, Kinderlehrer R, Haznedar MM, Shihabuddin L, Siever LJ: Three-dimensional analysis with MRI and PET of the size, shape, and function of the thalamus in the schizophrenia spectrum. Am J Psychiatry 1999; 156:1190-1199Google Scholar
15. Weinberger DR: Cell biology of the hippocampal formation in schizophrenia. Biol Psychiatry 1999; 45:395-402Crossref, Medline, Google Scholar
16. Krimer LS, Herman MM, Saunders RC, Boyd JC, Hyde TM, Carter JM, Kleinman JE, Weinberger DR: A qualitative and quantitative analysis of the entorhinal cortex in schizophrenia. Cereb Cortex 1997; 7:732-739Crossref, Medline, Google Scholar
17. Pakkenberg B: Pronounced reduction of total neuron number in mediodorsal thalamic nucleus and nucleus accumbens in schizophrenics. Arch Gen Psychiatry 1990; 47:1023-1028Google Scholar
18. Clinton SM, Haroutunian V, Davis KL, Meador-Woodruff JH: Altered expression of NMDA receptor-associated post-synaptic density proteins in the thalamus in schizophrenia. Proc Natl Acad Sci USA (in press)Google Scholar
19. Tamminga CA, Vogel M, Gao X, Lahti AC, Holcomb HH: The limbic cortex in schizophrenia: focus on the anterior cingulate. Brain Res Brain Res Rev 2000; 31:364-370Crossref, Medline, Google Scholar
20. Javitt DC, Zukin SR: Recent advances in the phencyclidine model of schizophrenia. Am J Psychiatry 1991; 148:1301-1308Google Scholar
21. Krystal JH, Karper LP, Seibyl JP, Freeman GK, Delaney R, Bremmer JD, Heninger GR, Bowers MB, Charney DS: Subanesthetic effects of the noncompetitive NMDA antagonist, ketamine, in humans: psychotomimetic, perceptual, cognitive and neuroendocrine responses. Arch Gen Psychiatry 1994; 51:199-214Crossref, Medline, Google Scholar
22. Krystal JH, D’Souza DC, Petrakis IL, Belger A, Berman RM, Charney DS, Abi-Saab W, Madonick S: NMDA agonists and antagonists as probes of glutamatergic dysfunction and pharmacotherapies in neuropsychiatric disorders. Harv Rev Psychiatry 1999; 7:125-143Crossref, Medline, Google Scholar
23. Krystal JH, Moghaddam B, Breier A, Goldman-Rakic PS, McElvey J: Glutamate, dopamine, the frontal cortex, and schizophrenia (abstract). Biol Psychiatry 1998; 43(suppl):60SGoogle Scholar
24. Anand A, Charney DS, Oren DA, Berman RM, Hu XS, Cappiello A, Krystal JH: Attenuation of the neuropsychiatric effects of ketamine with lamotrigine: support for hyperglutamatergic effects of N-methyl-d-aspartate receptor antagonists. Arch Gen Psychiatry 2000; 57:270-276Crossref, Medline, Google Scholar
25. Halberstadt AL: The phencyclidine-glutamate model of schizophrenia. Clin Neuropharmacol 1995; 18:237-249Crossref, Medline, Google Scholar
26. Lahti AC, Holcomb HH, Medoff DR, Tamminga CA: Ketamine activates psychosis and alters limbic blood flow in schizophrenia. Neuroreport 1995; 6:869-872Crossref, Medline, Google Scholar
27. Lahti AC, Koffel B, LaPorte D, Tamminga CA: Subanesthetic doses of ketamine stimulate psychosis in schizophrenia. Neuropsychopharmacology 1995; 13:9-19Crossref, Medline, Google Scholar
28. Olney JW, Farber NB: Glutamate receptor dysfunction and schizophrenia. Arch Gen Psychiatry 1995; 52:998-1007Google Scholar
29. Carlsson A, Waters N, Carlsson ML: Neurotransmitter interactions in schizophrenia—therapeutic implications. Biol Psychiatry 1999; 46:1388-1395Google Scholar
30. Nishikawa T, Takashima M, Toru M: Increased [3H] kainic acid binding in the prefrontal cortex in schizophrenia. Neurosci Lett 1983; 40:245-250Crossref, Medline, Google Scholar
31. Toru M, Watanabe S, Shibuya H, Nishikawa T, Noda K, Mitsushio H, Ichikawa H, Kurumaji A, Takashima M, Mataga N, Ogawa A: Neurotransmitters, receptors and neuropeptides in post-mortem brains of chronic schizophrenic patients. Acta Psychiatr Scand 1988; 78:121-137Crossref, Medline, Google Scholar
32. Kornhuber J, Mack Burkhardt F, Riederer P, Hebenstreit GF, Reynolds GP, Andrews HB, Beckmann H: [3H]MK-801 binding sites in postmortem brain regions of schizophrenic patients. J Neural Transm 1989; 77:231-236Crossref, Medline, Google Scholar
33. Byerley W, Bailey ME, Hicks AA, Riley BP, Darlison MG, Holik J, Hoff M, Umar F, Reimherr F, Wender P: Schizophrenia and GABAA receptor subunit genes. Psychiatr Genet 1995; 5:23-29Crossref, Medline, Google Scholar
34. Grimwood S, Slater P, Deakin JF, Hutson PH: NR2B-containing NMDA receptors are up-regulated in temporal cortex in schizophrenia. Neuroreport 1999; 10:461-465Crossref, Medline, Google Scholar
35. Simpson MD, Slater P, Deakin JF: Comparison of glutamate and gamma-aminobutyric acid uptake binding sites in frontal and temporal lobes in schizophrenia. Biol Psychiatry 1998; 44:423-427Crossref, Medline, Google Scholar
36. Longson D, Deakin JF, Benes FM: Increased density of entorhinal glutamate-immunoreactive vertical fibers in schizophrenia. J Neural Transm 1996; 103:503-507Crossref, Medline, Google Scholar
37. Meador-Woodruff JH, Haroutunian V, Davis KL, Watson SJ: NMDA receptor dysregulation in schizophrenic prefrontal cortex (abstract). Biol Psychiatry 1998; 43(suppl):83SGoogle Scholar
38. Akbarian S, Sucher N, Bradley D, Tafazzoli A, Trinh D, Hetrick W, Potkin S, Sandman C, Bunney W, Jones E: Selective alterations in gene expression for NMDA receptor subunits in prefrontal cortex of schizophrenics. J Neurosci 1996; 16:19-30Crossref, Medline, Google Scholar
39. Korpi ER, Kleinman JE, Goodman SI, Wyatt RJ: Neurotransmitter amino acids in post-mortem brains of chronic schizophrenic patients. Psychiatry Res 1987; 22:291-301Crossref, Medline, Google Scholar
40. Sherman AD, Hegwood TS, Baruah S, Waziri R: Deficient NMDA-mediated glutamate release from synaptosomes of schizophrenics. Biol Psychiatry 1991; 30:1191-1198Google Scholar
41. Deakin JF, Slater P, Simpson MD, Gilchrist AC, Skan WJ, Royston MC, Reynolds GP, Cross AJ: Frontal cortical and left temporal glutamatergic dysfunction in schizophrenia. J Neurochem 1989; 52:1781-1786Google Scholar
42. Gao X-M, Sakai K, Roberts RC, Conley RR, Dean B, Tamminga CA: Ionotropic glutamate receptors and expression of N-methyl-d-aspartate receptor subunits in subregions of human hippocampus: effects of schizophrenia. Am J Psychiatry 2000; 157:1141-1149Google Scholar
43. Simpson MDC, Slater P, Royston MC, Deakin JFW: Alterations in phencyclidine and sigma binding sites in schizophrenia brains: effects of disease process and neuroleptic medication. Schizophr Res 1992; 6:41-48Crossref, Google Scholar
44. Breier A: Cognitive deficit in schizophrenia and its neurochemical basis. Br J Psychiatry Suppl 1999; 37:16-18Medline, Google Scholar
45. Haroutunian V, Santucci AC: Pharmacological animal models of dementia, in Neurobiology of Mental Illness. Edited by Charney DS, Nestler EJ, Bunney BS. New York, Oxford University Press, 1999, pp 669-678Google Scholar
46. Olney JW, Newcomer JW, Farber NB: NMDA receptor hypofunction model of schizophrenia. J Psychiatr Res 1999; 33:523-533Crossref, Medline, Google Scholar
47. Anegawa NJ, Guttmann RP, Grant ER, Anand R, Lindstrom J, Lynch DR: N-Methyl-d-aspartate receptor mediated toxicity in nonneuronal cell lines: characterization using fluorescent measures of cell viability and reactive oxygen species production. Brain Res Mol Brain Res 2000; 77:163-175Crossref, Medline, Google Scholar
48. Trist DG: Excitatory amino acid agonists and antagonists: pharmacology and therapeutic applications. Pharm Acta Helv 2000; 74:221-229Crossref, Medline, Google Scholar
49. Hollmann M, Heinemann S: Cloned glutamate receptors, in Annual Review of Neuroscience. Edited by Cowan W, Shooter E, Stevens C, Thompson R. Palo Alto, Calif, Annual Reviews, 1994, pp 31-108Google Scholar
50. Gao XM, Tamminga CA: Human brain receptors, VIII: the distribution of ionotropic glutamate receptors in the human hippocampus (image). Am J Psychiatry 1994; 151:1271Link, Google Scholar
51. Huntley GW, Vickers JC, Morrison JH: Cellular and synaptic localization of NMDA and non-NMDA receptor subunits in neocortex: organizational features related to cortical circuitry, function and disease. Trends Neurosci 1994; 17:536-543Crossref, Medline, Google Scholar
52. Wenzel A, Scheurer L, Kunzi R, Fritschy JM, Mohler H, Benke D: Distribution of NMDA receptor subunit proteins NR2A, 2B, 2C and 2D in rat brain. Neuroreport 1995; 7:45-48Crossref, Medline, Google Scholar
53. Dodt HU, Frick A, Kampe K, Zieglgansberger W: NMDA and AMPA receptors on neocortical neurons are differentially distributed. Eur J Neurosci 1998; 10:3351-3357Google Scholar
54. Conti F, Minelli A, DeBiasi S, Melone M: Neuronal and glial localization of NMDA receptors in the cerebral cortex. Mol Neurobiol 1997; 14:1-18Crossref, Medline, Google Scholar
55. Rigby M, Le Bourdelles B, Heavens RP, Kelly S, Smith D, Butler A, Hammans R, Hills R, Xuereb JH, Hill RG, Whiting PJ, Sirinathsinghji DJ: The messenger RNAs for the N-methyl-d-aspartate receptor subunits show region-specific expression of different subunit composition in the human brain. Neuroscience 1996; 73:429-447Crossref, Medline, Google Scholar
56. Kaczmarek L, Kossut M, Skangiel-Kramska J: Glutamate receptors in cortical plasticity: molecular and cellular biology. Physiol Rev 1997; 77:217-255Crossref, Medline, Google Scholar
57. Kendrick SJ, Lynch DR, Pritchett DB: Characterization of glutamate binding sites in receptors assembled from transfected NMDA receptor subunits. J Neurochem 1996; 67:608-616Crossref, Medline, Google Scholar
58. Lynch DR, Lawrence JJ, Lenz S, Anegawa NJ, Dichter M, Pritchett DB: Pharmacological characterization of heterodimeric NMDA receptors composed of NR 1a and 2B subunits: differences with receptors formed from NR 1a and 2A. J Neurochem 1995; 64:1462-1468Google Scholar
59. Meador-Woodruff JH, Healy DJ: Glutamate receptor expression in schizophrenic brain. Brain Res Brain Res Rev 2000; 31:288-294Crossref, Medline, Google Scholar
60. Kennedy MB: The postsynaptic density at glutamatergic synapses. Trends Neurosci 1997; 20:264-268Crossref, Medline, Google Scholar
61. Ehlers MD: Synapse structure: glutamate receptors connected by the shanks. Curr Biol 1999; 9:R848-R850Google Scholar
62. Kornau HC, Schenker LT, Kennedy MB, Seeburg PH: Domain interaction between NMDA receptor subunits and the postsynaptic density protein PSD-95. Science 1995; 269:1737-1740Google Scholar
63. Christopherson KS, Hillier BJ, Lim WA, Bredt DS: PSD-95 assembles a ternary complex with the N-methyl-d-aspartic acid receptor and a bivalent neuronal NO synthase PDZ domain. J Biol Chem 1999; 274:27467-27473Google Scholar
64. Cattabeni F, Gardoni F, Di Luca M: Pathophysiological implications of the structural organization of the excitatory synapse. Eur J Pharmacol 1999; 375:339-347Crossref, Medline, Google Scholar
65. Sheng M, Pak DT: Glutamate receptor anchoring proteins and the molecular organization of excitatory synapses. Ann NY Acad Sci 1999; 868:483-493Crossref, Medline, Google Scholar
66. Hunt CA, Schenker LJ, Kennedy MB: PSD-95 is associated with the postsynaptic density and not with the presynaptic membrane at forebrain synapses. J Neurosci 1996; 16:1380-1388Google Scholar
67. Sattler R, Xiong Z, Lu WY, Hafner M, MacDonald JF, Tymianski M: Specific coupling of NMDA receptor activation to nitric oxide neurotoxicity by PSD-95 protein. Science 1999; 284:1845-1848Google Scholar
68. Aoki C, Bredt DS, Fenstemaker S, Lubin M: The subcellular distribution of nitric oxide synthase relative to the NR1 subunit of NMDA receptors in the cerebral cortex. Prog Brain Res 1998; 118:83-97Crossref, Medline, Google Scholar
69. Migaud M, Charlesworth P, Dempster M, Webster LC, Watabe AM, Makhinson M, He Y, Ramsay MF, Morris RG, Morrison JH, O’Dell TJ, Grant SG: Enhanced long-term potentiation and impaired learning in mice with mutant postsynaptic density-95 protein. Nature 1998; 396:433-439Crossref, Medline, Google Scholar
70. Davidson M, Harvey PD, Powchik P, Parrella M, White L, Knobler HY, Losonczy MF, Keefe RS, Katz S, Frecska E: Severity of symptoms in chronically institutionalized geriatric schizophrenic patients. Am J Psychiatry 1995; 152:197-207Link, Google Scholar
71. Harvey PD, Howanitz E, Parrella M, White L, Davidson M, Mohs RC, Hoblyn J, Davis KL: Symptoms, cognitive functioning, and adaptive skills in geriatric patients with lifelong schizophrenia: a comparison across treatment sites. Am J Psychiatry 1998; 155:1080-1086Google Scholar
72. Harvey PD, Parrella M, White L, Mohs RC, Davidson M, Davis KL: Convergence of cognitive and adaptive decline in late-life schizophrenia. Schizophr Res 1999; 35:77-84Crossref, Medline, Google Scholar
73. Purohit DP, Perl DP, Haroutunian V, Powchik P, Davidson M, Davis K: Alzheimer’s disease and related neurodegenerative diseases in elderly schizophrenic patients. Arch Gen Psychiatry 1998; 55:205-211Crossref, Medline, Google Scholar
74. Mirra SS, Heyman A, McKeel D, Sumi SM, Crain BJ, Brownlee LM, Vogel FS, Hughes JP, van Belle G, Berg L: The Consortium to Establish a Registry for Alzheimer’s Disease (CERAD), part II: standardization of the neuropathologic assessment of Alzheimer’s disease. Neurology 1991; 41:479-486Crossref, Medline, Google Scholar
75. Davis KL, Mohs RC, Marin DB, Purohit DP, Perl DP, Lantz M, Austin G, Haroutunian V: Cholinergic markers are not decreased in early Alzheimer’s disease. JAMA 1999; 281:1401-1406Google Scholar
76. Haroutunian V, Perl DP, Purohit DP, Marin DB, Khan K, Lantz M, Davis KL, Mohs RC: Regional distribution of neuritic plaques in nondemented elderly and cases of very mild Alzheimer’s disease. Arch Neurol 1998; 55:1185-1191Google Scholar
77. Rajkowska G, Goldman-Rakic PS: Cytoarchitectonic definition of prefrontal areas in the normal human cortex, 1: remapping of areas 9 and 46 using quantitative criteria. Cereb Cortex 1995; 5:307-322Crossref, Medline, Google Scholar
78. Daviss SR, Lewis DA: Local circuit neurons of the prefrontal cortex in schizophrenia: selective increase in the density of calbindin-immunoreactive neurons. Psychiatry Res 1995; 59:81-96Crossref, Medline, Google Scholar
79. Chomczynski P, Sacchi N: Single-step method of RNA isolation by acid guanidinium thiocyanate-phenol-chloroform extraction. Anal Biochem 1987; 162:156-159Crossref, Medline, Google Scholar
80. Vet JA, Majithia AR, Marras SA, Tyagi S, Dube S, Poiesz BJ, Kramer FR: Multiplex detection of four pathogenic retroviruses using molecular beacons. Proc Natl Acad Sci USA 1999; 96:6394-6399Google Scholar
81. Bonnet G, Tyagi S, Libchaber A, Kramer FR: Thermodynamic basis of the enhanced specificity of structured DNA probes. Proc Natl Acad Sci USA 1999; 96:6171-6176Google Scholar
82. Marras SA, Kramer FR, Tyagi S: Multiplex detection of single-nucleotide variations using molecular beacons. Genet Anal 1999; 14:151-156Crossref, Medline, Google Scholar
83. Tyagi S, Kramer FR: Molecular beacons: probes that fluoresce upon hybridization. Nat Biotechnol 1996; 14:303-308Crossref, Medline, Google Scholar
84. Higuchi R, Fockler C, Dollinger G, Watson R: Kinetic PCR analysis: real-time monitoring of DNA amplification reactions. Biotechnology (NY) 1993; 11:1026-1030Google Scholar
85. Ritter LM, Meador-Woodruff JH: Antipsychotic regulation of hippocampal dopamine receptor messenger RNA expression. Biol Psychiatry 1997; 42:155-164Crossref, Medline, Google Scholar
86. McNemar Q: Psychological Statistics. New York, John Wiley & Sons, 1969Google Scholar
87. Ozawa S, Kamiya H, Tsuzuki K: Glutamate receptors in the mammalian central nervous system. Prog Neurobiol 1998; 54:581-618Crossref, Medline, Google Scholar
88. Wang Y, TesFaye E, Yasuda RP, Mash DC, Armstrong DM, Wolfe BB: Effects of post-mortem delay on subunits of ionotropic glutamate receptors in human brain. Brain Res Mol Brain Res 2000; 80:123-131Crossref, Medline, Google Scholar
89. Ossowska K, Pietraszek M, Wardas J, Nowak G, Wolfarth S: Chronic haloperidol and clozapine administration increases the number of cortical NMDA receptors in rats. Naunyn Schmiedebergs Arch Pharmacol 1999; 359:280-287Crossref, Medline, Google Scholar
90. Tarazi FI, Florijn WJ, Creese I: Regulation of ionotropic glutamate receptors following subchronic and chronic treatment with typical and atypical antipsychotics. Psychopharmacology (Berl) 1996; 128:371-379Crossref, Medline, Google Scholar
91. Brene S, Messer C, Nestler EJ: Expression of messenger RNAs encoding ionotropic glutamate receptors in rat brain: regulation by haloperidol. Neuroscience 1998; 84:813-823Crossref, Medline, Google Scholar
92. Lynch DR, Anegawa NJ, Verdoorn T, Pritchett DB: N-Methyl-d-aspartate receptors: different subunit requirements for binding of glutamate antagonists, glycine antagonists, and channel-blocking agents. Mol Pharmacol 1994; 45:540-545Medline, Google Scholar
93. Sprengel R, Suchanek B, Amico C, Brusa R, Burnashev N, Rozov A, Hvalby O, Jensen V, Paulsen O, Andersen P, Kim JJ, Thompson RF, Sun W, Webster LC, Grant SG, Eilers J, Konnerth A, Li J, McNamara JO, Seeburg PH: Importance of the intracellular domain of NR2 subunits for NMDA receptor function in vivo. Cell 1998; 92:279-289Crossref, Medline, Google Scholar
94. Steigerwald F, Schulz TW, Schenker LT, Kennedy MB, Seeburg PH, Kohr G: C-Terminal truncation of NR2A subunits impairs synaptic but not extrasynaptic localization of NMDA receptors. J Neurosci 2000; 20:4573-4581Google Scholar
95. Wang C, Showalter VM, Hillman GR, Johnson KM: Chronic phencyclidine increases NMDA receptor NR1 subunit mRNA in rat forebrain. J Neurosci Res 1999; 55:762-769Crossref, Medline, Google Scholar
96. Conti F: Localization of NMDA receptors in the cerebral cortex: a schematic overview. Braz J Med Biol Res 1997; 30:555-560Crossref, Medline, Google Scholar