Mismatch Negativity Responses in Schizophrenia: A Combined fMRI and Whole-Head MEG Study
Abstract
OBJECTIVE: Mismatch negativity is an event-related brain response sensitive to deviations within a sequence of repetitive auditory stimuli. It is thought to reflect short-term sensory memory and is independent of higher-level cognitive processes. Mismatch negativity response is diminished in patients with schizophrenia. Little is known about the mechanisms of this decreased response, the contribution of the different hemispheres, and its locus of generation. METHOD: Patients with schizophrenia (N=12) and matched comparison subjects (N=12) were studied. A novel design to measure mismatch negativity responses to deviant auditory stimuli was generated by using the switching noises from the functional magnetic resonance imaging (fMRI) scanner, thus avoiding any interfering background sound. Stimuli included deviants of amplitude (9 dB lower) and duration (76 msec shorter) presented in a random sequence. The scanner noise was recorded and applied to the same subjects in a whole-head magnetoencephalography (MEG) device. Neuromagnetic and hemodynamic responses to the identical stimuli were compared between the patients and comparison subjects. RESULTS: As expected, neuromagnetic mismatch fields were smaller in the patient group. More specifically, a lateralization to the right for duration deviance was only found in comparison subjects. For the relative amplitude of the blood-oxygen-level-dependent signal (measured with fMRI), differences emerged in the secondary (planum temporale), but not primary (Heschl’s gyrus), auditory cortex. Duration deviants achieved a right hemispheric advantage only in the comparison group. A significantly stronger lateralization to the left was found for the deviant amplitude stimuli in the patients. CONCLUSIONS: The data support the view of altered hemispheric interactions in the formation of the short-term memory traces necessary for the integration of auditory stimuli. This process is predominantly mediated by the planum temporale (secondary auditory cortex). Altered interaction of regions within the superior temporal plane and across hemispheres could be in part responsible for language-mediated cognitive (e.g., verbal memory) and psychopathological (hallucinations, formal thought disorder) symptoms in schizophrenia.
Mismatch negativity is an event-related potential elicited when a sequence of unattended repetitive sounds is interrupted by a deviant stimulus (1). It is an electrophysiological correlate of sensory memory representation and can be observed even under distracted conditions. This preattentive response is generated at the level of the planum temporale and is likely to reflect N-methyl-d-aspartic acid (NMDA) channel current influx in cortical layers II and III as found in extracellular recordings in the monkey (2). In humans, a diminished mismatch negativity response following the administration of ketamine (an NMDA antagonist) has been described (3), consistent with NMDA/glutamate involvement in mismatch negativity generation.
The mismatch negativity response to duration deviants, usually recorded with EEG, is consistently reduced in patients with schizophrenia (4–7). In some studies of pitch deviants, this abnormality was not observed (8, 9) (for a review see reference 10). This impairment of sensory memory formation has also been described in unmedicated patients (4), first-degree relatives (11, 12), and in high-risk groups (13). Several studies have reported correlations between mismatch negativity amplitude and negative symptoms (4, 14), and it appears that the mismatch negativity response is particularly reduced over the left hemisphere in patients with schizophrenia (15–17). The mismatch negativity reduction seems not to be ameliorated by either typical (18) or atypical neuroleptics (19).
Mismatch negativity response in schizophrenia is of particular interest given the well-replicated findings of structural superior temporal gyrus abnormalities (20, 21) and the involvement of this brain area in core symptoms of the disease such as hallucinations (22, 23) and formal thought disorder (24). Furthermore, NMDA is suspected to be involved in the pathophysiology of schizophrenia (25). However, little is known about the mechanisms of decreased mismatch responses in schizophrenia, their etiological significance, or their precise locus of generation.
Functional magnetic resonance imaging (fMRI) allows precise localization of cerebral correlates of perceptual and cognitive operations. However, the noise generated by the scanner limits its application in the investigation of auditory processing. To avoid these shortcomings, we developed a method to record the hemodynamic analogue of mismatch responses by means of fMRI using the gradient switching noise as the sound source (26). The advantage of this technique is—besides its ease of use without external stimulation devices—that the sound source is part of the environment so that unattended processing prevails. This stimulation procedure does not yield the frontal activation (26) that suggests attentive processing as observed with other fMRI or positron emission tomography techniques. Moreover, the scanner noise can be recorded and applied within whole-head magnetoencephalography (MEG) to obtain comparable electrophysiological data.
The present study sought to further clarify the alteration of mismatch negativity response in patients with schizophrenia. Preattentive neuromagnetic and hemodynamic responses to amplitude and duration deviants within a series of standard noise bursts were recorded in a group of schizophrenia patients and comparison subjects. In a pilot study employing a similar paradigm in healthy subjects, we found lateralization to the right superior temporal plane only for the duration mismatch condition in MEG and fMRI (26). On the basis of these and findings with EEG (15–17), we hypothesized altered lateralization patterns on the level of the superior temporal plane in the patients for deviant duration stimuli.
Method
Subjects
Twelve inpatients with DSM-IV schizophrenia, all receiving stable doses of antipsychotic medication, were recruited from the Department of Psychiatry, University of Tübingen, Germany. Diagnosis was established by two independent psychiatrists through a clinical interview and review of the hospital charts. As seen in Table 1, a comparison group of healthy volunteers was matched with the patient group for sociodemographic and cognitive variables as well as nicotine use (per the Fagerstrom Index [27]). All subjects were right-handed (28). Only subjects with normal hearing per an audiometry test were included. Immediate memory recall and attention were assessed on the day of scanning by using the Digit Span (29) and the Continuous Performance Test (30). Subjects were clinically rated (by A.R.) with the Positive and Negative Syndrome Scale (31) on the day of scanning before collection of imaging data. Permission for the study was obtained from the local ethical committee. After complete description of the study to the subjects, written informed consent was obtained. Patients and comparison subjects received compensation (8 Euro/hour) for participating in the study.
Measurement of Magnetic Field Responses (MEG)
Auditory evoked magnetic fields were recorded by means of a 151-channel whole-head MEG (CTF System Inc., Vancouver, Canada) within an electromagnetically shielded room (sampling rate=250 Hz, anti-aliasing filtering=80 Hz). Recorded gradient switching noises served as auditory stimuli (duration=152 msec, amplitude about 80 dB). Two sessions comprising 450 sweeps each (sweep length=800 msec, prestimulus baseline=148 msec, interstimulus interval=810 msec) included 20% randomly interspersed deviants (about 90 of the 450 sweeps). The deviant stimuli were those with amplitude or duration reductions of 9 dB and 76 msec, respectively, which occurred with equal probability. During the entire session, subjects watched silent movies (Charlie Chaplin). They were naive with respect to the experimental paradigm.
Data analysis was fully scripted and automatized to prevent observer errors. Equivalent eye dipoles were used to control for artifacts (symmetric two-dipole model) by eliminating sweeps with eye-blinks characterized by moments >60 nAm. The remaining sweeps obtained from each subject were averaged. Source analysis relied on a simple-sphere head model comprising two tangential dipole currents minimizing residual variance. Dipole fitting was performed at the time point of maximum global field power within a time domain extending from 50 to 96 msec after stimulus onset. Calculation of the individual lead-field functions relied on dipole position and direction. These two linear combinations of the 151 channels maximize the signal-to-noise ratio of current estimation for the respective auditory cortex. The two components provide a separate measure of the time course of dipole strength in both hemispheres (32, 33). Mismatch responses were calculated as the difference waves between averaged responses to the deviants, on the one hand, and the last stimulus of the preceding series of standards, on the other. The last stimulus preceding the infrequent one was used because it is the “most frequent” one and produces the most reliable response. In order to minimize noise, data were weighted by using prestimulus variance as a reference. The rationale for this technique is given in detail elsewhere (34). Quantitative estimates of mismatch fields were obtained within a time window derived from the average mismatch time course. The center of gravity of the computed difference curves between 140 and 280 msec served as a measure of mismatch field latency.
MEG fields represent the sum of multiple signal and noise sources at separate locations. Consequently, intrasubject variability was considered an additive error term allowing for robust linear statistics. In each subject, the probability of the null hypothesis was derived by bootstrap procedures based on the corresponding regression model (35). To that means, the regression coefficients were obtained for 999 independent drawn samples to approximate to empirical distribution of the estimator (35). The probability of this distribution under the null hypothesis was expressed as a z score for each individual. The latter values were averaged across group to test whether the null hypothesis for all subjects could be rejected. Thus, no variance measure was required, avoiding sensitivity to outliers in small sample sizes. Moreover, relative to variance analytic methods, this nonparametric analogue of an analysis of variance procedure exhibits faster convergence and does not presuppose identical distribution families across subjects.
A lateralization score from the interval (–1, 1) was evaluated for each subject as (L–R)/(|L|+|R|), with L and R being the quantified mismatch responses over the left and right hemisphere, respectively.
The groups were compared with respect to activity summed over both hemispheres. This measure is comparable to frontally detected EEG mismatch negativity and is held to reflect the well-known finding of reduced mismatch reactions in schizophrenia (36). In addition, whole-head MEG allowed study of the lateralization score for each subject. In a bootstrapping procedure, the average of the patients was tested to differ from that of the comparison group. Data analysis was performed with Matlab 5 (Mathworks Inc., USA), using a significance level of p<0.05.
Measurement of Hemodynamic Responses (fMRI)
Magnetic resonance imaging was performed with a 1.5-T clinical MR scanner (Sonata, Siemens, Germany). First, a T1-weighted anatomical image (three-dimensional fast low-angle shots) was obtained for each subject. Subsequently, functional imaging was conducted. A single-shot, multi-echo echo planar imaging sequence applied a 64×64 matrix, achieving a readout time of 38 msec per echo (TE=26, 64, 120, and 140 msec, nonlinear sampling on sinusoidal gradient ramps, bandwidth=50 kHz, field of view=210×210 mm2, slice thickness=5 mm, slice gap=1 mm, voxel size=3.3×3.3×6 mm3). Acquisition of four echoes per slice (duration=152 msec) represented the frequent events, occurring at a stimulus onset asynchrony of 800 msec. The strength of the imaging gradients was lowered by a factor of 0.35 for amplitude deviants (–9 dB sound pressure level, 7.5% of the events). The sound of the encoding of two instead of four echoes yielded a duration mismatch (–76 msec, 7.5% of the events) (Figure 1). Sound pressure level of the gradient switching noise amounted to 94.5 dB at the head (about –28 dB dampening due to standard noise-canceling headphones). Four imaging slices were acquired covering the supratemporal plane and Heschl’s gyrus in parallel orientation to the Sylvian fissure. The number of stimuli (number of slice acquisitions) amounted to 512, i.e., 128 volumes with four slices each (TR=3,200 msec). Slices encoded during deviant events (15%) were discarded from statistical analysis. A multi-echo sequence was applied 1) to obtain acoustic stimulation of a suitable duration and 2) to increase the sensitivity to the blood-oxygen-level-dependent (BOLD) effect (37). As with the MEG experiment, subjects watched a silent movie and were naive with respect to the acoustic stimulation.
Analysis of the multi-echo MR data required preprocessing before application of standard statistical parametric mapping (SPM 99 [38]) procedures. Differential weighting of the four successive echoes optimized signal-to-noise or contrast-to-noise ratio, respectively. In order to maximize signal-to-noise ratio, each of the acquired echoes (i=1,…4) was weighted before averaging as follows: (exp[–TEi/80 msec])2, where TEi=echo time of ith echo. These images will be referred to as signal-to-noise ratio images. For optimal contrast-to-noise ratio of the BOLD effect, the echoes were averaged using the weight factors (TEi × exp[–TEi/65 msec])2 (contrast-to-noise ratio images). Realignment and normalization of images were performed by using the parameter estimates obtained from the signal-to-noise ratio images. Motion correction was restricted within the acquisition plane (39). Statistical parametric maps were obtained by applying a general linear model on the signal intensities of the normalized and smoothed (12 mm full width at half maximum Gauss-kernel) contrast-to-noise ratio images. The convolution of the bi-gamma function and the indicator function of the respective rare events served as reference vector for the paradigm, i.e., the modeled hemodynamic response function (Figure 1). Analysis was repeated for each slice separately applying the respective time delay. Then the calculated statistical maps were combined.
Regions of interest were defined on the anatomical template (Talairach space) in both the primary auditory cortex (±45, –16, 4 mm, Brodmann’s area 41) and secondary auditory cortex (±63, –21, 6 mm, Brodmann’s area 42). Percent signal changes were analyzed due to differing signal-to-noise ratios in the two groups (40). Lateralization scores were the differences between left and right hemispheric region of interest, weighted by the variance in the secondary auditory cortex. To maintain linear statistics, the difference measures were not normalized to the summed responses.
Statistics followed analysis of variance in a repeated-measure design. Statistical maps were corrected for multiple testing across the volume based on the Gaussian field theory (38) (p<0.05). Testing across the regions of interest was Bonferroni corrected (p<0.05).
Between-group differences were tested by using a t test (p<0.05, volume corrected).
Results
Magnetic Field Responses
One patient failed to complete the functional imaging sessions and was excluded from the data analysis. The remaining 11 patients participated in both MEG sessions. Dipole fits did not differ in P50m amplitude or lateralization between groups. In seven out of the 11 patients and 11 out of the 12 comparison subjects, a significant mismatch field was detected at the single-subject level (cutoff at p<0.05). Across both groups, deviants of amplitude (–9 dB) and duration (76 msec shorter) elicited reliable mismatch responses over both hemispheres, achieving high significance levels in both groups (Table 2 and Figure 2).
As depicted in Figure 3, a significant side difference emerged only in the comparison group, and only for duration deviants, as a right-hemispheric enhancement (–3.3 nA; z=3.0, p<0.001).
The mismatch responses summed across both hemispheres proved to be smaller in the patients for both amplitude deviants (z=2.5, p<0.05) and duration deviants (z=3.1, p<0.05). The lateralization scores (L–R)/(|L|+|R|) did not differ between the groups for amplitude deviants (mean score 0.03 versus 0.04) (z=6.3, p>0.20). As the most important finding, response to duration deviants was significantly more lateralized for the comparison subjects than for the schizophrenia patients (0.19 versus –0.16) (z=2.2, p<0.05).
Hemodynamic Responses
In both groups, the changes of amplitude and duration of the gradient switching noise elicited reliable hemodynamic responses in both hemispheres in the primary and secondary auditory cortex. A direct comparison of statistical parametric maps (volume corrected, extent ≥15 voxels) revealed a stronger response in the comparison group in the left auditory cortex for amplitude deviants (p<0.05) but not duration deviants (Figure 4).
Lateralization scores were derived from region-of-interest analyses in the primary and secondary auditory cortex (Figure 5). Amplitude changes elicited larger hemodynamic responses at the left side in both the schizophrenia patients (z=4.4, p<0.001) and comparison subjects (z=3.5, p<0.001). As a main result, the duration deviants achieved a right hemispheric advantage only in the comparison group (comparison subjects: z=18.7, p<0.001; schizophrenia patients: z=0.8, p=0.22).
Stronger lateralization patterns emerged in the patient group than in the comparison subjects for amplitude deviants (i.e., larger left-to-right scores in the patients than in the comparison subjects: z=2.5, p<0.01). No significant contrast was apparent for duration deviants, although the comparison subjects tended to have larger right-to-left scores than did the schizophrenia group (z=1.2, p=0.11).
Discussion
This study employed a novel design in a combined fMRI and MEG setting to compare cerebral responses to deviant auditory stimuli in patients with schizophrenia and matched comparison subjects. To circumvent the interfering background sound of the MRI scanner, its gradient switching noises were used as stimuli. The noise was recorded and applied in a whole-head MEG device. In the comparison group, the deviant stimuli evoked reliably right-lateralized mismatch MEG responses. The patients exhibited decreased responses and failed to show a lateralization effect in line with our hypothesis. In the fMRI setting, duration deviants elicited right lateralized signal increases in the planum temporale in the comparison subjects. The patients, in contrast, exhibited symmetric signal changes. The results of this study confirmed our earlier findings in the comparison subjects (26), and the patient data confirms our hypothesis of dysfunctional lateralization in this group.
The groups in our study were carefully matched for possible confounding effects such as age, gender, and nicotine use. Previous event-related potential studies have reported effects of smoking on P50 and P300 (41, 42), although influences on N100 have not been reported. Neuroleptic medication does not seem to influence mismatch negativity response (18, 19). Because of the size of our study group, the influence of gender on lateralization could not be calculated.
Although our mismatch stimuli might be slightly different in interstimulus interval or duration from standard EEG paradigms, they reliably evoked the well-known neuromagnetic responses in different control populations (26). Corroborating earlier findings of EEG studies (36, 43), the patients showed reduced mismatch fields to the duration as well as amplitude deviants. We could extend these findings and demonstrate that the decrease is at least in part explained by the missing right-hemispheric enhancement in the patients in the planum temporale, but not Heschl’s gyrus. In the only other MEG study investigating mismatch field in schizophrenia (16), such an altered lateralization was not present. However, those authors did not find the normal lateralization pattern to the right in comparison subjects. They used a MEG device that could record responses over one hemisphere only. The sequential comparison of the responses might increase variance and be influenced by a crosstalk from the unobserved auditory cortex. We used a whole-head scanner, which is more reliable in detecting lateralization differences.
Previously, it has been demonstrated that the mismatch negativity response has multiple generators within the superior temporal gyrus (44). The precise localization of its main component cannot be detected with current MEG methods. Using fMRI with its high spatial resolution, our results demonstrated that the hemodynamic equivalent of the mismatch field in the comparison subjects is in part located in the secondary auditory cortex (planum temporale), with a stronger signal change in the right superior temporal plane. Furthermore, a lateralization deficit similar to the mismatch field paradigm in the patients for the duration mismatch was detected. This finding stands in contrast to another fMRI study of mismatch negativity response in schizophrenia, in which standard oddball stimuli were presented via headphones (45). The authors found a smaller number of activated voxels in the superior temporal gyrus in the patients than in the comparison group in the mismatch but not the control condition. They did not find lateralization differences between groups, and the strongest activation was present in the Heschl’s gyrus (primary auditory cortex) in both groups. However, a confounding effect in their study may have been scanner noise, which is known to activate primary auditory regions (46, 47) and to mask the effects of stimulus properties. Another difference is that they employed blocks with deviants compared with blocks without deviants whereas we assessed directly the hemodynamic response to the single deviant events. Thus, lateralized abnormality might be masked by the difference in the activations to the blocks without deviants or specific to the distinct types of deviance detection.
The role of frontal areas in the generation of mismatch fields is still under debate. A number of studies found differences in mismatch responses between patients with schizophrenia and comparison subjects in the frontal (48–50), in the frontal and temporal (51, 52), or exclusively temporal (53–55) regions. Neither in our comparison nor in the patient group did we find responses in frontal areas. Differences in the technique applied and stimulus properties might be responsible for the different results in these studies. It has been proposed that the involvement of frontal areas in the generation of mismatch fields reflects attentive components (56, 57). The comparison with event-related potential recordings suggests that distinct networks participate in preattentive processing and target detection (58). Our paradigm allowed stimulation without attentive processing and thus little frontal contributions would be expected. Moreover, putative attentional deficits in patients would not have an influence in our paradigm. Thus, we focused on the auditory cortices, where we expected the most reliable differences between the groups. Further, our fMRI paradigm allowed only for a limited number of slices to be acquired, and the ventral regions of the frontal lobe were therefore not covered.
The BOLD response reflects differential hemodynamic activation integrated across seconds. Thus, neither early versus late components of a mismatch response, nor processing of a deviant versus its succeeding frequent stimulus, can be entirely separated. Amplitude changes modified responses in the primary auditory cortex due to deviant detection as well as adaptation effects, which cause primary, as well as secondary, auditory cortical responses. The effects of duration deviants on primary areas were minimal. Peak sound pressure level remains the same for the duration deviants whereas amplitude deviants may change the adaptation level. Therefore, the first louder sound may elicit adaptation phenomena in primary auditory areas. We suggest that an integration of activity in the primary and secondary (59) auditory cortices of both hemispheres is necessary for a normal mismatch response. A temporal desynchronization of the process is likely to underlie the deviant mismatch field response in our patients (60). The differences in mismatch fields and BOLD response between the groups in our study might be due to alterations in synaptic connections, pyramidal cell distribution, or connectivity between cortical columns. This might cause reduced stimulus-induced phase locking in the auditory cortex in the patients (61) and thus altered mismatch fields. The fMRI and MEG experiments yielded distinct effects for the topography and lateralization patterns. As a possible explanation, later components might be integrated into the BOLD response, whereas the mismatch field is restricted to the given temporal domain. Nonetheless, no differences emerged in the temporally resolved MEG for the response latencies between the groups. The different lateralization patterns in the fMRI and MEG data may thus reflect differences in temporal integration and synchronization processes between patients and comparison subjects.
Mismatch negativity elicitation is attributed to the presence of short-term memory traces. Templates are formed in the auditory cortex to encode the frequent stimulus elements. These memory traces usually fade within 5–10 seconds (62). For mismatch negativity to occur, an integrative process, consisting of an analysis of the afferent sound (deviant) and its comparison to the memory trace (frequent stimulus) has to occur (63). Impaired language processing in childhood is one of the few known risk factors for the later development of schizophrenia (64). Furthermore, some of the main symptoms in schizophrenia, such as formal thought disorder, auditory hallucinations, and concretism, involve language functions. We previously demonstrated a reversed lateralization pattern of cerebral activation in the superior temporal gyrus in patients with schizophrenia during processing of linguistic context (65) and production of fluent speech (66). The production of thought-disordered speech correlated negatively with signal changes in the posterior superior temporal gyrus (Wernicke’s area [24]).
In the current study, we have shown that language-related dysfunction in schizophrenia may be present already at the early stages of auditory processing of simple stimuli, and not only at stages involving higher-order semantic processing. This dysfunction involves an impaired lateralization of cortical activation in the secondary auditory cortex (planum temporale). Synchronized activity as measured with MEG was more affected than the metabolic changes measured by fMRI. Potential volume differences in the superior temporal gyrus between patient and comparison groups in our study were corrected by the fMRI analysis method. In line with our findings are results from studies using structural MRI. They found a reduction in the volume of the left planum temporale (secondary auditory cortex) but not Heschl’s gyrus (primary auditory cortex) in patients (67–69) (for review see references 70, 71). We therefore could provide a potential link between altered planum temporale morphology in schizophrenia and its functional consequences, i.e., disrupted preattentive acoustic processing.
In conclusion, we found altered neural responses to basic sound processing at the level of the planum temporale in a group of patients with schizophrenia in line with morphological changes in this region. Although the underlying pathology remains elusive, it might be related to deficient NMDA-dependent neurotransmission (3) and impaired synaptic connectivity (72) and thus reduced stimulus-induced phase locking of cortically evoked activity. The superior temporal gyrus is a crucial area in the generation of language-related symptoms in schizophrenia such as formal thought disorder (24) and auditory hallucinations (23), so this region warrants attention in future research.
![]() |
![]() |
Received April 14, 2003; revision received Aug. 5, 2003; accepted Aug. 7, 2003. From the University of Tübingen Departments of Psychiatry, Neuroradiology, and Neurology and the MEG Center and Institute of Medical Psychology and Behavioral Neurobiology, University of Tübingen. Address reprint requests to Dr. Kircher, Department of Psychiatry, University of Tübingen, Osianderstr. 24, D-72076 Tübingen, Germany; [email protected] (e-mail). Supported by a grant from the University of Tübingen (fortuene number 727-0-0) and the German Research Foundation (DFG SFB 550). The authors thank Maike Borutta for technical assistance.
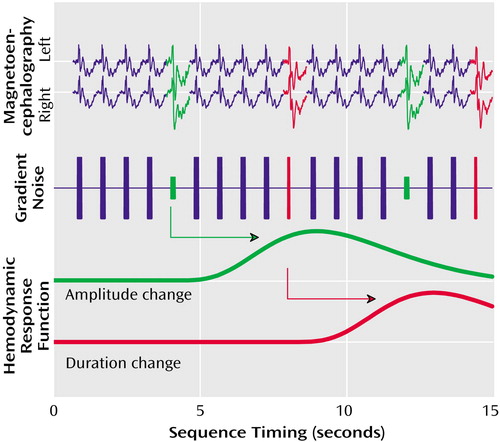
Figure 1. Experimental Design Scheme for Measurement of Electrophysiological Responses to Deviant Auditory Stimuli in Schizophrenia Patients and Healthy Comparison Subjectsa
aThe gradient switching noises elicited by the MRI scanner provided identical acoustic stimuli for both the fMRI and MEG experiments. MEG measured magnetic field responses to deviations in amplitude (green) and duration (red) in the left and right hemisphere. The hemodynamic response function, measured with fMRI, represents blood-oxygen-level-dependent signal changes following each deviant event.
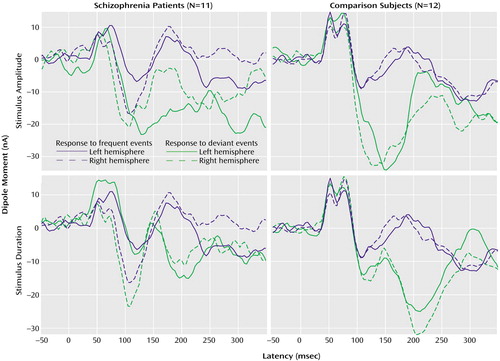
Figure 2. Magnetic Field Responses to Frequent and Deviant Auditory Stimuli in Schizophrenia Patients and Healthy Comparison Subjectsa
aResponses were measured with whole-head MEG. The gradient switching noises elicited by the MRI scanner provided acoustic stimuli. The frequent stimuli were switching noises of 152 msec and 80 dB; the deviant stimuli were those with duration and amplitude reductions of 76 msec and 9 dB, respectively.
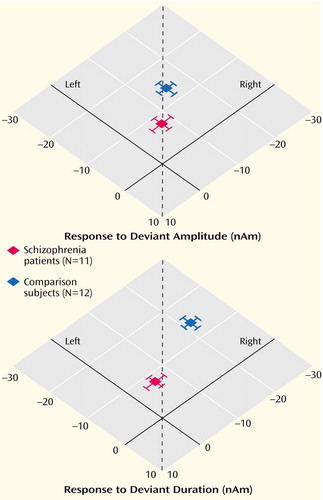
Figure 3. Lateralization of Magnetic Field Responses to Deviant Auditory Stimuli in Patients With Schizophrenia and Healthy Comparison Subjectsa
aResponses were measured with whole-head MEG. Deviant stimuli were those gradient switching noises elicited by the MRI scanner with duration and amplitude reductions of 76 msec and 9 dB, respectively, from the frequent stimuli. The dotted lines mark the position of symmetric responses.
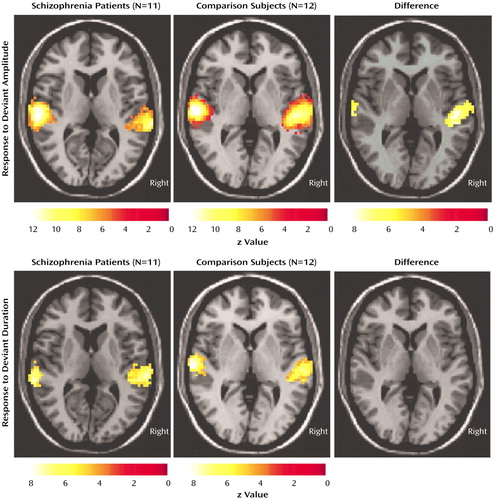
Figure 4. Hemodynamic Responses to Deviant Auditory Stimuli in Patients With Schizophrenia and Healthy Comparison Subjectsa
aBlood-oxygen-level-dependent signal changes measured with fMRI. Deviant stimuli were those gradient switching noises elicited by the MRI scanner with duration and amplitude reductions of 76 msec and 9 dB, respectively, from the frequent stimuli.
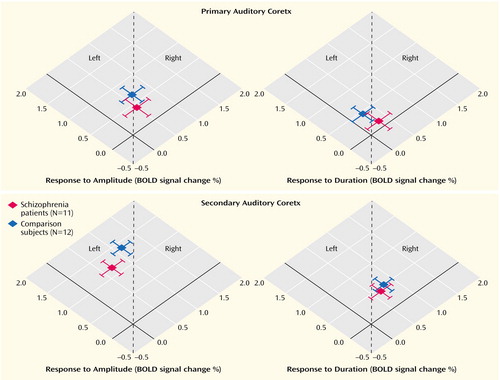
Figure 5. Lateralization of Hemodynamic Responses to Deviant Auditory Stimuli in the Primary and Secondary Auditory Cortices of Patients With Schizophrenia and Healthy Comparison Subjectsa
aBlood-oxygen-level-dependent (BOLD) signal changes were measured with fMRI. Deviant stimuli were those gradient switching noises elicited by the MRI scanner with amplitude and duration reductions of 9 dB and 76 msec, respectively, from the frequent stimuli. The dotted lines mark the position of symmetric responses.
1. Naatanen R, Gaillard AW, Mantysalo S: Early selective-attention effect on evoked potential reinterpreted. Acta Psychol (Amst) 1978; 42:313–329Crossref, Medline, Google Scholar
2. Javitt DC, Schroeder CE, Steinschneider M, Arezzo JC, Vaughan H-GJ: Demonstration of mismatch negativity in the monkey. Electroencephalogr Clin Neurophysiol 1992; 83:87–90Crossref, Medline, Google Scholar
3. Umbricht D, Schmid L, Koller R, Vollenweider FX, Hell D, Javitt DC: Ketamine-induced deficits in auditory and visual context-dependent processing in healthy volunteers: implications for models of cognitive deficits in schizophrenia. Arch Gen Psychiatry 2000; 57:1139–1147Crossref, Medline, Google Scholar
4. Catts SV, Shelley A-M, Ward PB, Liebert B, McConaghy N, Andrews S, Michie PT: Brain potential evidence for an auditory sensory memory deficit in schizophrenia. Am J Psychiatry 1995; 152:213–219Link, Google Scholar
5. Todd J, Michie PT, Budd TW, Rock D, Jablensky AV: Auditory sensory memory in schizophrenia: inadequate trace formation? Psychiatry Res 2000; 96:99–115Crossref, Medline, Google Scholar
6. Javitt DC, Shelley AM, Silipo G, Lieberman JA: Deficits in auditory and visual context-dependent processing in schizophrenia: defining the pattern. Arch Gen Psychiatry 2000; 57:1131–1137Crossref, Medline, Google Scholar
7. Umbricht D, Koller R, Schmid L, Skrabo A, Grubel C, Huber T, Stassen H: How specific are deficits in mismatch negativity generation to schizophrenia? Biol Psychiatry 2003; 53:1120–1131Crossref, Medline, Google Scholar
8. O’Donnell BF, Hokama H, McCarley RW, Smith RS, Salisbury DF, Mondrow E, Nestor PG, Shenton ME: Auditory ERPs to non-target stimuli in schizophrenia: relationship to probability, task-demands, and target ERPs. Int J Psychophysiol 1994; 17:219–231Crossref, Medline, Google Scholar
9. Kathmann N, Wagner M, Rendtorff N, Engel RR: Delayed peak latency of the mismatch negativity in schizophrenics and alcoholics. Biol Psychiatry 1995; 37:754–757Crossref, Medline, Google Scholar
10. Michie PT, Budd TW, Todd J, Rock D, Wichmann H, Box J, Jablensky AV: Duration and frequency mismatch negativity in schizophrenia. Clin Neurophysiol 2000; 111:1054–1065Crossref, Medline, Google Scholar
11. Jessen F, Fries T, Kucharski C, Nishimura T, Hoenig K, Maier W, Falkai P, Heun R: Amplitude reduction of the mismatch negativity in first-degree relatives of patients with schizophrenia. Neurosci Lett 2001; 309:185–188Crossref, Medline, Google Scholar
12. Michie PT, Innes-Brown H, Todd J, Jablensky AV: Duration mismatch negativity in biological relatives of patients with schizophrenia spectrum disorders. Biol Psychiatry 2002; 52:749–758Crossref, Medline, Google Scholar
13. Schreiber H, Stolz-Born G, Kornhuber HH, Born J: Event-related potential correlates of impaired selective attention in children at high risk for schizophrenia. Biol Psychiatry 1992; 32:634–651Crossref, Medline, Google Scholar
14. Salisbury DF, Shenton ME, Griggs BA, Bonner-Jackson A, McCarley RW: Mismatch negativity in chronic schizophrenia and first-episode schizophrenia. Arch Gen Psychiatry 2002; 59:686–694Crossref, Medline, Google Scholar
15. Hirayasu Y, Shenton ME, Salisbury DF, Dickey CC, Fischer IA, Mazzoni P, Kisler T, Arakaki H, Kwon JS, Anderson JE, Yurgelun-Todd D, Tohen M, McCarley R: Lower left temporal lobe MRI volumes in patients with first-episode schizophrenia compared with psychotic patients with first-episode affective disorder and normal subjects. Am J Psychiatry 1998; 155:1384–1391Link, Google Scholar
16. Kreitschmann-Andermahr I, Rosburg T, Meier T, Volz HP, Nowak H, Sauer H: Impaired sensory processing in male patients with schizophrenia: a magnetoencephalographic study of auditory mismatch detection. Schizophr Res 1999; 35:121–129Crossref, Medline, Google Scholar
17. Javitt DC, Doneshka P, Grochowski S, Ritter W: Impaired mismatch negativity generation reflects widespread dysfunction of working memory in schizophrenia. Arch Gen Psychiatry 1995; 52:550–558Crossref, Medline, Google Scholar
18. Pekkonen E, Hirvonen J, Ahveninen J, Kahkonen S, Kaakkola S, Huttunen J, Jaaskelainen IP: Memory-based comparison process not attenuated by haloperidol: a combined MEG and EEG study. Neuroreport 2002; 13:177–181Crossref, Medline, Google Scholar
19. Umbricht D, Javitt D, Novak G, Bates J, Pollack S, Lieberman J, Kane J: Effects of clozapine on auditory event-related potentials in schizophrenia. Biol Psychiatry 1998; 44:716–725Crossref, Medline, Google Scholar
20. Shenton ME, Kikinis R, Jolesz FA, Pollak SD, LeMay M, Wible CG, Hokama H, Martin J, Metcalf D, Coleman M, et al: Abnormalities of the left temporal lobe and thought disorder in schizophrenia: a quantitative magnetic resonance imaging study. N Engl J Med 1992; 327:604–612Crossref, Medline, Google Scholar
21. Falkai P, Bogerts B, Schneider T, Greve B, Pfeiffer U, Pilz K, Gonsiorzcyk C, Majtenyi C, Ovary I: Disturbed planum temporale asymmetry in schizophrenia: a quantitative post-mortem study. Schizophr Res 1995; 14:161–176Crossref, Medline, Google Scholar
22. Dierks T, Linden DE, Jandl M, Formisano E, Goebel R, Lanfermann H, Singer W: Activation of Heschl’s gyrus during auditory hallucinations. Neuron 1999; 22:615–621Crossref, Medline, Google Scholar
23. Shergill SS, Brammer MJ, Williams SCR, Murray RM, McGuire PK: Mapping auditory hallucinations in schizophrenia using functional magnetic resonance imaging. Arch Gen Psychiatry 2000; 57:1033–1038Crossref, Medline, Google Scholar
24. Kircher TTJ, Liddle PF, Brammer MJ, Williams SCR, Murray RM, McGuire PK: Neural correlates of formal thought disorder in schizophrenia. Arch Gen Psychiatry 2001; 58:769–774Crossref, Medline, Google Scholar
25. Javitt DC, Steinschneider M, Schroeder CE, Arezzo JC: Role of cortical N–methyl-d–aspartate receptors in auditory sensory memory and mismatch negativity generation: implications for schizophrenia. Proc Natl Acad Sci USA 1996; 93:11962–11967Crossref, Medline, Google Scholar
26. Mathiak K, Rapp A, Kircher TTJ, Grodd W, Hertrich I, Weiskopf N, Lutzenberger W, Ackermann H: Mismatch response to randomized gradient switching noise as reflected by fMRI and whole-head magnetoencephalography. Hum Brain Mapp 2002; 16:190–195Crossref, Medline, Google Scholar
27. Heatherton TF, Kozlowski LT, Frecker RC, Fagerstrom KO: The Fagerstrom Test for Nicotine Dependence: a revision of the Fagerstrom Tolerance Questionnaire. Br J Addict 1991; 86:1119–1127Crossref, Medline, Google Scholar
28. Annett M: Classification of hand preference by association analysis. Br J Psychol 1970; 61:303–321Crossref, Medline, Google Scholar
29. Wechsler D: Wechsler Adult Intelligence Scale—Revised (WAIS-R UK). London, Psychological Corp, 1981Google Scholar
30. Weintraub S, Mesulam MM: Mental state assessment of young and elderly adults in behavioral neurology, in Principles of Behavioral Neurology. Edited by Mesulam MM. Philadelphia, Davis, 1985, pp 101–103Google Scholar
31. Kay SR, Fiszbein A, Opler LA: The Positive and Negative Syndrome Scale (PANSS) for schizophrenia. Schizophr Bull 1987; 13:261–276Crossref, Medline, Google Scholar
32. Mathiak K, Hertrich I, Lutzenberger W, Ackermann H: Preattentive processing of consonant vowel syllables at the level of the supratemporal plane: a whole-head magnetoencephalography study. Brain Res Cogn Brain Res 1999; 8:251–257Crossref, Medline, Google Scholar
33. Mathiak K, Hertrich I, Lutzenberger W, Ackermann H: Encoding of temporal speech features (formant transients) during binaural and dichotic stimulus application: a whole-head magnetoencephalography study. Brain Res Cogn Brain Res 2000; 10:125–131Crossref, Medline, Google Scholar
34. Mathiak K, Hertrich I, Lutzenberger W, Ackermann H: Functional cerebral asymmetries of pitch processing during dichotic stimulus application: a whole-head magnetoencephalography study. Neuropsychologia 2002; 40:585–593Crossref, Medline, Google Scholar
35. Efron B, Tibshirani RJ: An Introduction to the Bootstrap. New York, London, Chapman & Hall, 1993Google Scholar
36. Michie PT: What has MMN revealed about the auditory system in schizophrenia? Int J Psychophysiol 2001; 42:177–194Crossref, Medline, Google Scholar
37. Posse S, Wiese S, Gembris D, Mathiak K, Kessler C, Grosse Ruyken ML, Elghahwagi B, Richards T, Dager SR, Kiselev VG: Enhancement of BOLD-contrast sensitivity by single-shot multi-echo functional MR imaging. Magn Reson Med 1999; 42:87–97Crossref, Medline, Google Scholar
38. Friston KJ, Turner R: SPM 99. http://www.fil.ion.ucl.ac.uk/spm/spm99.htmlGoogle Scholar
39. Mathiak K, Posse S: Evaluation of motion and realignment for functional magnetic resonance imaging in real time. Magn Reson Med 2001; 45:167–171Crossref, Medline, Google Scholar
40. Callicott JH, Ramsey NF, Tallent K, Bertolino A, Knable MB, Coppola R, Goldberg T, van Gelderen P, Mattay VS, Frank JA, Moonen CT, Weinberger DR: Functional magnetic resonance imaging brain mapping in psychiatry: methodological issues illustrated in a study of working memory in schizophrenia. Neuropsychopharmacology 1998; 18:186–196Crossref, Medline, Google Scholar
41. Crawford HJ, McClain-Furmanski D, Castagnoli NJ, Castagnoli K: Enhancement of auditory sensory gating and stimulus-bound gamma band (40 Hz) oscillations in heavy tobacco smokers. Neurosci Lett 2002; 317:151–155Crossref, Medline, Google Scholar
42. Anokhin AP, Vedeniapin AB, Sirevaag EJ, Bauer LO, O’Connor SJ, Kuperman S, Porjesz B, Reich T, Begleiter H, Polich J, Rohrbaugh JW: The P300 brain potential is reduced in smokers. Psychopharmacology (Berl) 2000; 149:409–413Crossref, Medline, Google Scholar
43. Javitt DC, Grochowski S, Shelley AM, Ritter W: Impaired mismatch negativity (MMN) generation in schizophrenia as a function of stimulus deviance, probability, and interstimulus/interdeviant interval. Electroencephalogr Clin Neurophysiol 1998; 108:143–153Crossref, Medline, Google Scholar
44. May P, Tiitinen H, Ilmoniemi RJ, Nyman G, Taylor JG, Naatanen R: Frequency change detection in human auditory cortex. J Comput Neurosci 1999; 6:99–120Crossref, Medline, Google Scholar
45. Wible CG, Kubicki M, Yoo S-S, Kacher DF, Salisbury DF, Anderson MC, Shenton ME, Hirayasu Y, Kikinis R, Jolesz FA, McCarley RW: A functional magnetic resonance imaging study of auditory mismatch in schizophrenia. Am J Psychiatry 2001; 158:938–943Link, Google Scholar
46. Hall DA, Summerfield AQ, Goncalves MS, Foster JR, Palmer AR, Bowtell RW: Time-course of the auditory BOLD response to scanner noise. Magn Reson Med 2000; 43:601–606Crossref, Medline, Google Scholar
47. Talavage TM, Edmister WB, Ledden PJ, Weisskoff RM: Quantitative assessment of auditory cortex responses induced by imager acoustic noise. Hum Brain Mapp 1999; 7:79–88Crossref, Medline, Google Scholar
48. Sato Y, Yabe H, Todd J, Michie P, Shinozaki N, Sutoh T, Hiruma T, Nashida T, Matsuoka T, Kaneko S: Impairment in activation of a frontal attention-switch mechanism in schizophrenic patients. Biol Psychol 2003; 62:49–63Crossref, Medline, Google Scholar
49. Baldeweg T, Klugman A, Gruzelier JH, Hirsch SR: Impairment in frontal but not temporal components of mismatch negativity in schizophrenia. Int J Psychophysiol 2002; 43:111–122Crossref, Medline, Google Scholar
50. Kasai K, Okazawa K, Nakagome K, Hiramatsu K, Hata A, Fukuda M, Honda M, Miyauchi M, Matsushita M: Mismatch negativity and N2b attenuation as an indicator for dysfunction of the preattentive and controlled processing for deviance detection in schizophrenia: a topographic event-related potential study. Schizophr Res 1999; 35:141–156Crossref, Medline, Google Scholar
51. Kasai K, Nakagome K, Itoh K, Koshida I, Hata A, Iwanami A, Fukuda M, Kato N: Impaired cortical network for preattentive detection of change in speech sounds in schizophrenia: a high-resolution event-related potential study. Am J Psychiatry 2002; 159:546–553Link, Google Scholar
52. Hirayasu Y, Potts GF, O’Donnell BF, Kwon JS, Arakaki H, Akdag SJ, Levitt JJ, Shenton ME, McCarley RW: Auditory mismatch negativity in schizophrenia: topographic evaluation with a high-density recording montage. Am J Psychiatry 1998; 155:1281–1284Link, Google Scholar
53. Pekkonen E, Katila H, Ahveninen J, Karhu J, Huotilainen M, Tuhonen J: Impaired temporal lobe processing of preattentive auditory discrimination in schizophrenia. Schizophr Bull 2002; 28:467–474Crossref, Medline, Google Scholar
54. Youn T, Park HJ, Kim JJ, Kim MS, Kwon JS: Altered hemispheric asymmetry and positive symptoms in schizophrenia: equivalent current dipole of auditory mismatch negativity. Schizophr Res 2003; 59:253–260Crossref, Medline, Google Scholar
55. Park HJ, Kwon JS, Youn T, Pae JS, Kim JJ, Kim MS, Ha KS: Statistical parametric mapping of LORETA using high density EEG and individual MRI: application to mismatch negativities in schizophrenia. Hum Brain Mapp 2002; 17:168–178Crossref, Medline, Google Scholar
56. Hall DA, Haggard MP, Akeroyd MA, Palmer AR, Summerfield AQ, Elliott MR, Gurney EM, Bowtell RW: “Sparse” temporal sampling in auditory fMRI. Hum Brain Mapp 1999; 7:213–223Crossref, Medline, Google Scholar
57. Yoshiura T, Zhong J, Shibata DK, Kwok WE, Shrier DA, Numaguchi Y: Functional MRI study of auditory and visual oddball tasks. Neuroreport 1999; 10:1683–1688Crossref, Medline, Google Scholar
58. Opitz B, Mecklinger A, von Cramon DY, Kruggel F: Combining electrophysiological and hemodynamic measures of the auditory oddball. Psychophysiology 1999; 36:142–147Crossref, Medline, Google Scholar
59. Mustovic H, Scheffler K, Di Salle F, Esposito F, Neuhoff JG, Hennig J, Seifritz E: Temporal integration of sequential auditory events: silent period in sound pattern activates human planum temporale. Neuroimage 2003; 20:429–434Crossref, Medline, Google Scholar
60. Kwon JS, O’Donnell BF, Wallenstein GV, Greene RW, Hirayasu Y, Nestor PG, Hasselmo ME, Potts GF, Shenton ME, McCarley RW: Gamma frequency-range abnormalities to auditory stimulation in schizophrenia. Arch Gen Psychiatry 1999; 56:1001–1005Crossref, Medline, Google Scholar
61. Winterer G, Ziller M, Dorn H, Frick K, Mulert C, Wuebben Y, Herrmann WM, Coppola R: Schizophrenia: reduced signal-to-noise ratio and impaired phase-locking during information processing. Clin Neurophysiol 2000; 111:837–849Crossref, Medline, Google Scholar
62. Naatanen R, Jiang D, Lavikainen J, Reinikainen K, Paavilainen P: Event-related potentials reveal a memory trace for temporal features. Neuroreport 1993; 5:310–312Crossref, Medline, Google Scholar
63. Naatanen R, Winkler I: The concept of auditory stimulus representation in cognitive neuroscience. Psychol Bull 1999; 125:826–859Crossref, Medline, Google Scholar
64. Jones P, Rodgers B, Murray R, Marmot M: Child development risk factors for adult schizophrenia in the British 1946 birth cohort. Lancet 1994; 344:1398–1402Crossref, Medline, Google Scholar
65. Kircher TTJ, Bullmore ET, Brammer MJ, Williams SCR, Broome MR, Murray RM, McGuire PK: Differential activation of temporal cortex during sentence completion in schizophrenic patients with and without formal thought disorder. Schizophr Res 2001; 50:27–40Crossref, Medline, Google Scholar
66. Kircher TTJ, Liddle PF, Brammer MJ, Williams SCR, Murray RM, McGuire PK: Reversed lateralisation of temporal activation during speech production in thought disordered patients with schizophrenia. Psychol Med 2002; 32:439–449Crossref, Medline, Google Scholar
67. Kwon J-S, McCarley R-W, Hirayasu Y, Anderson J-E, Fischer I-A, Kikinis R, Jolesz F-A, Shenton M-E: Left planum temporale volume reduction in schizophrenia. Arch Gen Psychiatry 1999; 56:142–148Crossref, Medline, Google Scholar
68. Sumich A, Chitnis XA, Fannon DG, O’Ceallaigh S, Doku VC, Falrowicz A, Marshall N, Matthew VM, Potter M, Sharma T: Temporal lobe abnormalities in first-episode psychosis. Am J Psychiatry 2002; 159:1232–1234Link, Google Scholar
69. Goldstein JM, Seidman LJ, O’Brien LM, Horton NJ, Kennedy DN, Makris N, Caviness VS Jr, Faraone SV, Tsuang MT: Impact of normal sexual dimorphisms on sex differences in structural brain abnormalities in schizophrenia assessed by magnetic resonance imaging. Arch Gen Psychiatry 2002; 59:154–164Crossref, Medline, Google Scholar
70. Shapleske J, Rossell SL, Woodruff PW, David AS: The planum temporale: a systematic, quantitative review of its structural, functional and clinical significance. Brain Res Brain Res Rev 1999; 29:26–49Crossref, Medline, Google Scholar
71. Shenton ME, Dickey CC, Frumin M, McCarley RW: A review of MRI findings in schizophrenia. Schizophr Res 2001; 49:1–52Crossref, Medline, Google Scholar
72. Garey LJ, Ong WY, Patel TS, Kanani M, Davis A, Mortimer AM, Barnes TR, Hirsch SR: Reduced dendritic spine density on cerebral cortical pyramidal neurons in schizophrenia. J Neurol Neurosurg Psychiatry 1998; 65:446–453Crossref, Medline, Google Scholar