Dysfunction of Early-Stage Visual Processing in Schizophrenia
Abstract
OBJECTIVE: Schizophrenia is associated with deficits in higher-order processing of visual information. This study evaluated the integrity of early visual processing in order to evaluate the overall pattern of visual dysfunction in schizophrenia. METHOD: Steady-state visual-evoked potential responses were recorded over the occipital cortex in patients with schizophrenia and in age- and sex-matched comparison volunteers. Visual-evoked potentials were obtained for stimuli composed of isolated squares that were modulated sinusoidally in luminance contrast, number of squares, or chromatic contrast in order to emphasize magnocellular or parvocellular visual pathway activity. RESULTS: Responses of patients to magnocellular-biased stimuli were significantly lower than those of comparison volunteers. These lower response levels were observed in conditions using both low luminance contrast and large squares that biased processing toward the magnocellular pathway. In contrast, responses to stimuli that biased processing toward the parvocellular pathway were not significantly different between schizophrenia patients and comparison volunteers. A significant interaction of group and stimulus type was observed in the condition using low luminance contrast. CONCLUSIONS: These findings suggest a dysfunction of lower-level visual pathways, which was more prominent for magnocellular than parvocellular biased stimuli. The magnocellular pathway helps in orienting toward salient stimuli. A magnocellular pathway deficit could contribute to higher-level visual cognitive deficits in schizophrenia.
Schizophrenia is commonly considered to be a neurocognitive disorder (1–4). Perceptual dysfunction, however, may also play a prominent pathophysiological role and may provide important clues regarding schizophrenia’s underlying etiology (5–9). In the visual system, patients with schizophrenia have greater visual thresholds (10–13) and greater sensitivity to backward masking (6, 10–15), which may be attributable to hyperactivity within transient visual channels (16–19). Patients with schizophrenia also have impaired motion perception, spatial localization, and eye tracking (7, 8, 20–25), as well as difficulty detecting simple visual stimuli (26, 27). The pattern of deficits in visual processing in patients with schizophrenia suggests dysfunction even at the earliest stages of cortical processing. Event-related potential responses provide an objective index of neurophysiological processing at the level of the sensory cortex. The present study used a neurophysiological approach to evaluate mechanisms underlying dysfunction of early-stage visual processing in schizophrenia.
Over the past decade, significant advances have been made in understanding the visual system on a neurophysiological level. The retino-geniculo-cortical visual system is divided into magnocellular and parvocellular pathways (28, 29). The magnocellular and parvocellular pathways begin in the retina and project, by means of the lateral geniculate nucleus, to the striate cortex. Properties of the magnocellular pathway may allow it to be involved in initial detection and segregation of objects from the background, while properties of the parvocellular pathway may allow it to code details of objects (28, 29). For instance, the magnocellular pathway is specialized for processing rapidly changing stimuli, while the parvocellular pathway is specialized for processing slowly changing, clearly defined patterns or objects (28, 29).
Differential properties of the magnocellular and parvocellular pathway cells make it possible to evaluate the relative role of magnocellular and parvocellular pathways in schizophrenia. Magnocellular cells are more sensitive than parvocellular cells to stimuli with low luminance contrast but saturate more quickly as a function of increasing luminance contrast (29). In fact, the cortical neurons that receive parvocellular input do not respond below 8% contrast (30). Thus, stimuli with low luminance contrast bias processing toward the magnocellular pathway, while stimuli that are modulated around a high standing luminance contrast level bias processing toward the parvocellular pathway. Magnocellular and parvocellular cells also show differential sensitivity to size. Magnocellular cells are activated vigorously by stimulus elements that are relatively large (i.e., of low spatial frequency), whereas parvocellular cells are activated more strongly by stimulus elements that are relatively small (i.e., of high spatial frequency) (28, 29). In addition, magnocellular cells respond to objects with low contrast and to the movement of these objects in the visual field, while parvocellular cells are relatively insensitive to movement (28). Finally, magnocellular cells are not highly responsive to chromatic (color) contrast, while parvocellular cells are (28, 29). As a result of these differences in neuronal sensitivity, magnocellular pathway responses can be differentiated from parvocellular pathway responses by means of psychophysical or neurophysiological approaches. The differential properties of magnocellular and parvocellular cells contribute to the psychophysical properties of transient and sustained visual channels but do not strictly translate into these channels.
Electrophysiological studies (31, 32) examining low-level visual function in schizophrenia have produced varied results. For example, Schwartz et al. (33) found deficits in responses to stimuli with low spatial frequency, which could be indicative of dysfunction in the magnocellular pathway. In contrast, Jibiki et al. (34) found no deficits but a lack of variability in response amplitudes of schizophrenic patients when number of squares was varied. The authors of some psychophysical studies (20, 25, 26, 35) have also relied primarily on manipulations of spatial frequency to bias responding and have also produced conflicting results. To examine magnocellular and parvocellular function in schizophrenia, steady-state visual-evoked potentials were obtained not only by using spatial frequency but also by using luminance and color contrast to emphasize activity in the magnocellular or parvocellular pathways.
Method
Participants
Twenty-four male outpatients meeting DSM-IV criteria for schizophrenia at the New York Campus of the Department of Veterans Affairs New York Harbor Health Care System provided written informed consent after the procedures had been fully explained. Diagnoses were obtained by means of chart review, consultation with the treating psychiatrist, and the Structured Clinical Interview for DSM-IV (SCID). Patients were excluded if they met criteria for alcohol or substance abuse in the past 5 years or had any neurological disorder that might affect performance. All participants underwent an ophthalmological examination and were excluded from the study if they had any ocular disease. Any individuals with deficits of color vision were also excluded. Color vision was assessed with the Ishihara test (36), the Farnsworth Panel D-15 test (37), and the Lanthony Desaturated Panel D-15 test (38). The first assesses deficits of red-green color vision, and the latter two assess deficits of red-green and blue-yellow color vision. Four patients were taking atypical antipsychotics (olanzapine or risperidone), two were medication free, and 18 were receiving typical antipsychotic medications. Eight were taking anticholinergics. The mean chlorpromazine-equivalent dose was 752.1 mg/day (range=0–2500).
Twenty-two male comparison volunteers, age matched to the patients, provided written informed consent after the procedures had been fully explained. Comparison volunteers with a history of psychiatric disorder or substance abuse, as assessed by the SCID, with neurological or ophthalmologic disorder, or deficits in color vision (red-green or blue-yellow) were excluded. All participants had 20/30-corrected visual acuity or better.
The patient and comparison groups did not differ significantly in age (patients, mean=49.2 years, SD=11.5; comparison subjects, mean=50.4 years, SD=9.1), although socioeconomic status, as measured with the Hollingshead scale, was significantly lower for the patients (mean=24.7, SD=9.8, N=22) than for the comparison subjects (mean=56.7, SD=10.5, N=20) (t=10.14, df=40, p<0.001). Scores for negative symptoms, positive symptoms, and general psychopathology on the Positive and Negative Syndrome Scale (39) for patients were mean=15.3, SD=5.7, mean=14.1, SD=3.9, and mean=31.4, SD=7.1, respectively.
Apparatus
Patterns were generated by means of a VENUS system (Neuroscientific Corporation, Farmingdale, N.Y.) on a red-green-blue monitor with a frame rate of ∼119 Hz (noninterlaced). This same system was also used to record and analyze EEG activity synchronized frame by frame to the stimulus display.
Steady-state visual-evoked potentials were elicited by means of a sweep technique. The method for obtaining sweep visual-evoked potentials was first introduced by Regan (40) and was later advanced by several investigators (41–43). This technique differs from the more commonly used technique involving transient visual-evoked potential responses in that stimuli are presented rapidly (e.g., >3 Hz), and therefore, successive responses overlap in time. When these records are analyzed in the frequency domain (i.e., by means of Fourier analysis), stimulus-elicited activity can be differentiated from background EEG. In the method that involves sweep steady-state visual-evoked potentials, one stimulus parameter (e.g., spatial frequency or contrast) is varied in brief, discrete steps while contrast is modulated within each step to yield an entire response function in a several-second run (41–43). The sweep technique allows for rapid assessment of visual function in observers with limited attention spans.
Stimuli
Viewing distance was 114 cm, and the stimulus field subtended 8°×8° the visual angle. A small fixation dot was placed in the center of the display. Sweep visual-evoked potentials were elicited by means of sinusoidal temporal modulation of an array of isolated squares on a steady background (44) (Figure 1). Spatial frequency and depth of modulation were varied in separate runs, and stimulus parameters were manipulated to selectively drive parallel visual pathways (45–47). For achromatic conditions, the luminance of the squares was modulated below that of the static background, producing negative contrast (dark squares). Background luminance for these achromatic conditions was 100 candela/m2. For the chromatic condition, red squares appeared on a yellow background. Luminance of the yellow background was 60 cd/m2.
Three types of stimulus conditions were used: luminance contrast, number of squares, and chromatic contrast. During a single run of parameters, luminance contrast, spatial frequency, or chromatic contrast were varied in discrete steps. Each step was 120 display frames (∼1 sec) in duration; the contrast or spatial frequency increased in octave steps throughout the run.
Luminance contrast
Response to changes in luminance contrast were obtained under stimulus conditions designed to emphasize magnocellular or parvocellular activity (45). In the condition emphasizing the magnocellular pathway, depth of modulation was varied between 0% and 32%, with a standing luminance contrast level (pedestal) that equaled the depth of modulation, so that stimuli always appeared then disappeared, returning to 0% contrast. Depth of modulation is the percent of luminance modulation. Thus, if the depth of modulation was 8% and was presented around a standing contrast level of 8%, maximum luminance contrast would be 16%, and minimum would be 0%. To emphasize the parvocellular pathway, depth of modulation was again varied between 0% and 32%, but stimuli were presented around a standing luminance contrast level of 48%, so that contrast never went below 16%. Thus, in this condition, the squares never disappeared.
In both conditions, stimuli consisted of a 32×32 array of isolated squares (square size=15 min of arc of visual angle). The initial step in the sweep had 0% depth of modulation, which was followed by steps of 1%, 2%, 4%, 8%, 16%, and 32% depths of modulation. Each of the seven steps was presented for ∼1 sec. Stimuli were presented at a rate of 12 times per second.
Number of squares
In the condition emphasizing the magnocellular pathway, depth of modulation was fixed at 8% and presented around a standing luminance contrast level of 8%. Thus, stimuli again appeared then disappeared. In the condition emphasizing the parvocellular pathway, a high-contrast standing luminance contrast level of 48% was again used, and depth of modulation was 16%. Thus, the maximum luminance contrast was 64%, the minimum was 32%, and the stimuli never disappeared. In both conditions, the numbers of squares were 2×2, 4×4, 8×8, 16×16, 32×32, 64×64, and 128×128 (square size was 240–3.75 min of arc of visual angle). Fewer squares correspond to larger square size. The largest of the seven square sizes in the sweep was presented first and the smallest last. Each of the seven steps was presented for ∼1 sec. Stimuli were presented at a rate of six times per second.
Chromatic contrast
Stimuli were isolated red squares (32×32) on a yellow background that appeared then disappeared. Isoluminance is the point at which there is no difference in luminance between two stimuli that differ in chromatic contrast. The electrophysiological technique of Zemon et al. (46) was used, and isoluminance was estimated through manipulation of the ratio of red and green guns of the red-green-blue display monitor. Signals from the two guns were modulated in counterphase (i.e., 180° out of phrase with respect to one another). The red-green ratio that produced the lowest amplitude response in the visual evoked potential and/or the ratio in which the phase reached a peak or a trough was used as the isoluminant point (for detailed methodology, see reference 47).
Once isoluminance was determined, the chromatic contrast sweep was run. The appearance and disappearance of the isoluminant red squares involved counterphase modulation of the red and green guns with the blue gun set to zero luminance. Chromatic contrast was defined by the depth of modulation of the red gun. The green gun was always modulated in counterphase with the red gun at a depth of modulation used to yield isoluminance for each participant. The depths of modulation of the red gun were 8%, 20%, 40%, 60%, 80%, and 100%. The lowest depth of modulation was presented first in the sweep and the highest last. Each of the six steps was presented for ∼1 sec. Stimuli were presented at a rate of six times per second.
Visual-Evoked Potentials
Visual-evoked potentials were recorded from the occipital site relative to the vertex site reference by means of gold-cup electrodes placed on the midline of the scalp (48). The ground was placed at the parietal site.
The raw EEG was amplified (by 20,000), filtered with a bandpass of 0.1–100 Hz, and digitized at a rate of four times the monitor frame rate (∼476 Hz). Each EEG epoch (∼1 sec duration) corresponded to a single step in the sweep run. Frequency analysis was performed for each epoch. Amplitude and phase measures at the fundamental (stimulus) frequency (6 or 12 Hz) were extracted by means of a discrete Fourier transform (see references 40 and 44 for detailed methodology). Each sweep condition was run 10 times. Ten sweep functions were averaged, and signal-to-noise ratios for each mean epoch were calculated by means of the T2circ statistic (43, 49). Signal-to-noise ratios represent the amplitude divided by the radius of the circular 95% confidence region. By definition, a signal-to-noise ratio of 1.0 or more indicates a significant visual-evoked potential response at an alpha level of 0.05. A signal-to-noise ratio of less than 1.0 indicates that the visual-evoked potential response is not significantly different from ongoing EEG noise.
Procedure
The participants were refracted for the viewing distance and tested monocularly after they were light adapted to the mean luminance of the display for several minutes. They were seated in a dimly lit room and instructed to fixate on the small dot placed in the center of the display during each run. The experimenter (I.S.) monitored the gaze of each participant during each run to ensure steady fixation. Any runs in which gaze was unsteady (the participant looked away from center or blinked excessively) were rejected, and those runs were then repeated. In addition, if the EEG trace (displayed in the upper region of the monitor screen) contained sizable deflections from baseline or other noise activity, the run was rejected and then repeated. Brief rest periods were provided between runs while data were stored.
Statistical Analysis
Demographic comparisons were analyzed between groups by means of two-tailed t tests. Five repeated measures analyses of variance (ANOVAs) (using the multivariate analog), each with one between-group factor (group) and one within-group repeated measure (depth of modulation, number of squares, or chromatic contrast) were used to compare groups on the three tasks eliciting visual-evoked potentials (luminance contrast, number of squares, or chromatic contrast). In addition, two repeated measures ANOVAs (using the multivariate analog) (luminance contrast or number of squares) were performed with one between-group measure (group) and two within-group repeated measures (depth of modulation or number of squares and stimulus type [emphasizing magnocellular or parvocellular pathways]) to evaluate potential differential deficits within the magnocellular and parvocellular pathways. Post hoc t tests were applied where the ANOVAs revealed significant main effects or interactions. Data were analyzed for normality by means of the Kolmogorov-Smirnov test and for equality of variance by using Levene’s test. No deviations from normality or equality of variance were observed. Data were missing for one patient each in the conditions involving luminance contrast and number of squares.
Results
Luminance Contrast
In the condition emphasizing the magnocellular pathway, signal-to-noise ratios exceeding background noise (i.e., signal-to-noise ratios of 1.0 or more) were obtained at 8% depth of modulation for both groups (Figure 2, left). Furthermore, for both groups, curves rose steeply and then saturated at 16% depth of modulation. The patients with schizophrenia showed significantly lower signal-to-noise ratios than the comparison group at all points at which signal exceeded noise (Figure 2, left). Signal-to-noise ratios for patients were approximately 50% of comparison values. ANOVAs conducted across all levels of depth of modulation showed a significant group effect (F=5.7, df=1, 43, p=0.02). When only points above noise were considered, an ANOVA also showed a significant group effect (F=7.6, df=1, 43, p=0.009).
For the condition emphasizing the parvocellular pathway, signal-to-noise ratios exceeding background noise were also obtained at 8% depth of modulation for both groups (Figure 2, right). In contrast to results from the magnocellular condition, curves increased linearly and did not saturate. There was no significant difference between the patient group and the comparison group in the luminance contrast condition emphasizing the parvocellular pathway (Figure 2, right). Group effects were not significant, regardless of whether all levels of depth of modulation were used (F=1.0, df=1, 43, p=0.34) or only points with signal-to-noise ratios exceeding noise (F=0.9, df=1, 43, p=0.34).
A repeated measures ANOVA of within-group factors of stimulus type (emphasizing magnocellular or parvocellular pathways) and depth of modulation and the between-group factor of diagnostic group demonstrated a significant interaction of group and stimulus type (Figure 2).
Number of Squares
The curves obtained in the condition emphasizing the magnocellular pathway peaked at a lower fundamental spatial frequency (i.e., smaller number of squares) than those obtained in the condition emphasizing the parvocellular pathway and making use of a high standing luminance contrast level, which is consistent with the differential sensitivity of the magnocellular and parvocellular pathways. For the condition emphasizing the magnocellular pathway, the group of patients with schizophrenia showed significantly lower signal-to-noise ratios than the comparison group when numbers of squares were 16×16 and 32×32 (Figure 3, left). Signal-to-noise ratios were lower (32% to 49%) than comparison values. In contrast, there was no significant difference between the patient group and the comparison group in the condition emphasizing the parvocellular pathway and number of squares (Figure 3, right). No significant interaction of group and stimulus type was found (F=1.9, df=1, 43, p=0.18).
Chromatic Contrast
The curves obtained during the chromatic contrast sweep were approximately linear, as would be expected from a parvocellular pathway function. Although the patient group showed numerically lower signal-to-noise ratios than the comparison group, no significant differences were found between the groups (Figure 4).
Discussion
This study examined the mechanisms underlying visual-processing deficits in schizophrenia by use of visual-evoked potentials. Signal-to-noise ratios for visual-evoked potentials for patients with schizophrenia were significantly lower than those of comparison volunteers in conditions that biased processing toward the magnocellular pathway but not in conditions that biased processing toward the parvocellular pathway, suggesting selective magnocellular pathway dysfunction.
In this study, two stimulus manipulations were performed to differentially activate the magnocellular and parvocellular pathways. First, luminance contrast was modulated with stimuli appearing then disappearing or with a high standing luminance contrast level. Second, spatial frequency (number of squares) was modulated at a fixed luminance contrast. Of the two manipulations, the best separation between magnocellular and parvocellular functions was found in the condition varying luminance contrast. The shape of the curves was consistent with those seen in single-cell recording studies of monkey lateral geniculate nuclei (29). In our study as well as in monkey studies, curves rose steeply at low luminance contrast, and saturation of the response occurred at about 16%. In both manipulations, robust differences were seen between the patient group and the comparison group in conditions emphasizing magnocellular but not parvocellular pathway function. A significant interaction of group and stimulus type (magnocellular versus parvocellular), moreover, was observed in the condition varying luminance contrast, which supports the concept of a differential magnocellular versus parvocellular pathway dysfunction. The chromatic contrast test results, emphasizing the parvocellular pathway, did not differ significantly between groups.
Previous studies examining early visual-processing deficits in schizophrenia have typically used only spatial frequency manipulations and have produced conflicting results. For example, in psychophysical studies, Schwartz and colleagues (27) and Schwartz and Winstead (35) demonstrated decreased contrast sensitivity (i.e., higher thresholds) to moving, but not stationary, low spatial frequency gratings (striped patterns). Slaghuis (26) found decreased contrast sensitivity to both moving and stationary low spatial frequency gratings. Chen et al. (20), however, did not find decreased contrast sensitivity to either moving or stationary low spatial frequency gratings. With regard to high spatial frequency gratings, decreased contrast sensitivity was found for stationary and moving high spatial frequency gratings in one study (26), whereas Schwartz and colleagues (27) and Schwartz and Winstead (35) found decreased contrast sensitivity to moving but not stationary high spatial frequency gratings. Electrophysiological studies have also contained conflicting results. Schwartz et al. (33) showed impaired generation of transient visual-evoked potentials in response to stimuli with low, but not high, spatial frequencies. Jibiki et al. (34) used steady-state visual-evoked potentials and found that comparison volunteers had greater amplitude responses to larger than smaller spatial elements but that the responses of patients did not vary. These studies support the concept that early visual processing in schizophrenia may be impaired, but they have not been consistently able to segregate the deficit in terms of dysfunction in magnocellular versus parvocellular pathways.
The finding in the present study of a deficit in the magnocellular pathway is consistent with the results from a large body of literature demonstrating deficits in backward masking in schizophrenia (6, 10–15). In this task, participants are presented with a target stimulus (e.g., a letter) followed by a high-energy mask (e.g., a field of Xs). A mask presented shortly after the target interrupts target processing, leading to the phenomenon of backward masking. In this task, patients with schizophrenia, asked to identify a first (target) stimulus followed by a second (masking) stimulus, need longer interstimulus intervals between target and mask than comparison volunteers to successfully identify the target stimulus. The magnocellular and parvocellular pathways correspond somewhat to the classical psychophysically defined transient and sustained visual channels. It has been postulated that backward masking deficits in schizophrenia are due to overactivity of the transient system (16–19). The present finding localizes deficits to within the magnocellular pathway, although it suggests the existence of a decreased, rather than increased, response.
Dysfunction of the magnocellular pathway may also account for other well-described aspects of neurophysiological dysfunction in schizophrenia. For example, the magnocellular pathway projects predominantly to the dorsal cortical stream (i.e., parietal lobe), which codes motion perception and spatial localization (28, 50). Patients with schizophrenia consistently show deficits in performing tasks that tap function in the dorsal stream, including velocity discrimination, coherent motion, spatial localization, and trajectory tasks (7, 18, 20–24). Attempts to demonstrate a dysfunction of the magnocellular system by using a psychophysical approach were unsuccessful in at least one of these studies (20). In contrast, the present study demonstrates robust dysfunction of the magnocellular system by using neurophysiological measures. The magnocellular system or dorsal stream pathway plays a prominent role in the control of eye movements (20, 51, 52). The present results, therefore, are also consistent with the finding of dysfunctional eye movement in schizophrenia (21, 22, 25).
Finally, deficits of the magnocellular pathway may also explain dysfunction even within object-recognition areas of the parvocellular pathway. The parvocellular pathway projects predominantly to the ventral cortical stream (i.e., temporal lobe), which codes object identification and chromatic contrast (28, 50). Although the dense direct magnocellular projections are to the dorsal stream, there are numerous points at which magnocellular inputs have access to the ventral stream (28). Since information transfer through the magnocellular pathway is generally faster, a crucial role of the magnocellular pathway may be to modulate and/or prime the ventral pathway (53). It is well known that patients with schizophrenia have prolonged thresholds for the recognition of letters (10–13), a task that is usually considered to be mediated predominantly by structures in the ventral stream (28, 50). However, Schwartz et al. (54) recently showed that when letters were composed of moving dots, patients with schizophrenia showed deficits of letter identification only at high, not low, dot velocity. Since the magnocellular pathway mediates the processing of rapidly moving stimuli, this finding suggests that deficits of letter recognition in schizophrenia may be secondary to dysfunction of the magnocellular pathway.
A limitation of this study is that 22 of the 24 patients were receiving antipsychotic medications at the time of testing. Thus, a medication effect cannot be excluded. Furthermore, dopamine is present in the retina, and alterations in dopamine can potentially affect contrast sensitivity (55). However, there is no reason to expect that such effects are selective for the function of magnocellular versus parvocellular pathways. Further, Jibiki et al. (56) found no effect of acute haloperidol administration on the generation of steady-state visual-evoked potentials. Similarly, deficits of visual backward masking have been observed in both medicated and unmedicated patients (11, 15, 57). In the present study, the two unmedicated patients had steady-state visual-evoked potential values lower than the mean of the patient group as a whole. The deficit of visual-evoked potentials is probably also not due to attentional dysfunction, since the patients with schizophrenia showed selective dysfunction in magnocellular versus parvocellular pathways.
In conclusion, our results demonstrate lower-level visual-processing deficits in schizophrenia that are referable primarily to the magnocellular pathway. Because the magnocellular pathway projects predominantly to the dorsal stream, deficits in functioning in the magnocellular pathway would be expected to lead to dysfunction in the dorsal stream, as has been observed consistently in schizophrenia (7, 8, 18–24). Because of magnocellular inputs to the ventral stream, a deficit of the magnocellular pathway might also contribute to ventral stream object recognition and letter identification deficits. In addition, a deficit in the magnocellular pathway, which is the faster-responding visual pathway, could produce a primary deficit in orienting and responding to stimuli. Thus, deficits in function in the magnocellular pathway may explain widespread cognitive and attentional deficits in schizophrenia. At present, mechanisms underlying a selective deficit in the magnocellular pathway are unclear. More work is necessary to determine the etiology of dysfunction in the magnocellular pathway, as well as the extent to which deficits of the magnocellular pathway are causal with regard to higher-level deficits and symptoms.
Presented in part at the 37th annual meeting of the American College of Neuropsychopharmacology, Las Croabas, Puerto Rico, Dec. 14–18, 1998, and at the seventh International Congress on Schizophrenia, Santa Fe, N.Mex., April 17–21, 1999. Received June 23, 2000; revision received Nov. 13, 2000; accepted Jan. 11, 2001. From the New York Campus of the Department of Veterans Affairs New York Harbor Health Care System, New York; the Departments of Psychiatry and Ophthalmology, New York University Medical Center, New York; the Nathan Kline Institute for Psychiatric Research; the Psychology Department, Hunter College of the City University of New York, New York; and the Ferkauf Graduate School of Psychology at Yeshiva University, Bronx, N.Y. Address reprint requests to Dr. Butler, Nathan Kline Institute for Psychiatric Research, 140 Old Orangeburg Rd., Orangeburg, NY 10962; [email protected] (e-mail). Funded in part by a VA Merit Review grant to Dr. Butler. The authors thank Drs. Erica Duncan, Ira Jasser, and Eric Peselow and the staff of the Day Unit at the New York Campus of the Department of Veterans Affairs New York Harbor Health Care System for referring patients for participation and also Dr. Elaine C. Hall for comments on a draft of this article.
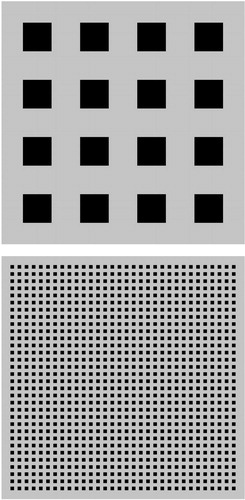
Figure 1. Examples of Stimuli Composed of Isolated Squares Used to Test Visual Processing in Patients With Schizophreniaa
aOn the top are fewer (4×4) and on the bottom are greater (32×32) numbers of isolated squares. These stimuli were used for two of the seven steps in the test involving increasing numbers of squares. The luminance contrast shown is quite high and represents the maximum luminance contrast used in the condition with a high standing luminance contrast level (pedestal).
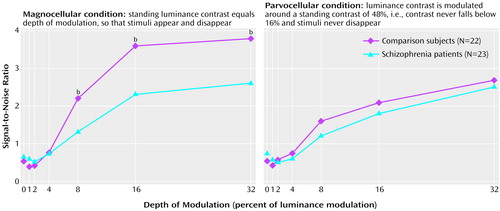
Figure 2. Signal-to-Noise Ratios for Patients With Schizophrenia and Comparison Volunteers in Test Conditions Using Luminance Contrast to Emphasize Magnocellular and Parvocellular Visual Pathwaysa
aSignificant interaction between group and stimulus type (magnocellular versus parvocellular) (F=5.0, df=1, 43, p=0.03).
bSignificant difference between groups (t=2.32–2.64, df=43, p<0.05).
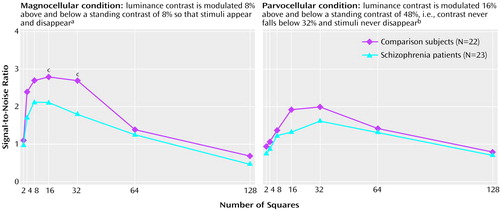
Figure 3. Signal-to-Noise Ratios for Patients With Schizophrenia and Comparison Volunteers in Test Conditions Using Number of Squares to Emphasize Magnocellular and Parvocellular Visual Pathways
aSignificant group effect (F=5.5, df=1, 43, p=0.02).
bNonsignificant group effect (F=2.4, df=1, 43, p=0.13).
cSignificant difference between groups (t=2.02–2.49, df=43, p<0.05).
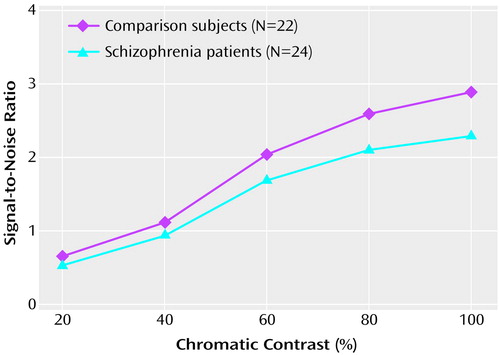
Figure 4. Signal-to-Noise Ratios for Patients With Schizophrenia and Comparison Volunteers in a Test Condition Using Chromatic Contrast at Isoluminance to Emphasize the Parvocellular Visual Pathwaya
aFor chromatic contrast, isolated red squares were presented on a yellow background with increasing percents of chromatic contrast. Isoluminance is the point at which there is no difference in luminance between two stimuli that differ in chromatic contrast.
1. Goldman-Rakic PS: Working memory dysfunction in schizophrenia. J Neuropsychiatry Clin Neurosci 1994; 6:348-357Crossref, Medline, Google Scholar
2. Goldberg TE, Gold JM: Neurocognitive functioning in patients with schizophrenia: an overview, in Psychopharmacology: The Fourth Generation of Progress. Edited by Bloom FE, Kupfer DJ. New York, Raven Press, 1995, pp 1245-1257Google Scholar
3. Weinberger DR, Gallhofer B: Cognitive function in schizophrenia. Int Clin Psychopharmacol 1997; 12(suppl 4):S29-S36Google Scholar
4. Green MF: Schizophrenia From a Neurocognitive Perspective. Boston, Allyn & Bacon, 1998Google Scholar
5. Adler LE, Freedman R, Ross RG, Olincy A, Waldo MC: Elementary phenotypes in the neurobiological and genetic study of schizophrenia. Biol Psychiatry 1999; 46:8-18Crossref, Medline, Google Scholar
6. Braff DL, Saccuzzo DP, Geyer MA: Information processing dysfunctions in schizophrenia: studies of visual backward masking, sensorimotor gating, and habituation, in Handbook of Schizophrenia, vol 5. Edited by Steinhauer SR, Gruzelier JH, Zubin J. New York, Elsevier, 1991, pp 303-334Google Scholar
7. Cadenhead KS, Serper Y, Braff DL: Transient versus sustained visual channels in the visual backward masking deficits of schizophrenia patients. Biol Psychiatry 1998; 43:132-138Crossref, Medline, Google Scholar
8. Malaspina D, Wray AD, Friedman JH, Amador X, Yale S, Hasan A, Gorman JM, Kaufmann CA: Odor discrimination deficits in schizophrenia: association with eye movement dysfunction. J Neuropsychiatry Clin Neurosci 1994; 6:273-278Crossref, Medline, Google Scholar
9. Javitt DC, Liederman E, Cienfuegos A, Shelley AM: Panmodal processing imprecision as a basis for dysfunction of transient memory storage systems in schizophrenia. Schizophr Bull 1999; 25:763-775Crossref, Medline, Google Scholar
10. Weiner RU, Opler LA, Kay SR, Merriam AE, Papouchis N: Visual information processing in positive, mixed, and negative schizophrenic syndromes. J Nerv Ment Dis 1990; 187:616-626Crossref, Google Scholar
11. Butler PD, Harkavy-Friedman JM, Amador XF, Gorman JM: Backward masking in schizophrenia: relationship to medication status, neuropsychological functioning, and dopamine metabolism. Biol Psychiatry 1996; 40:295-298Crossref, Medline, Google Scholar
12. Saccuzzo DP, Braff DL: Information-processing abnormalities: trait- and state-dependent components. Schizophr Bull 1986; 12:447-459Crossref, Medline, Google Scholar
13. Slaghuis WL, Bakker VJ: Forward and backward visual masking of contour by light in positive- and negative-symptom schizophrenia. J Abnorm Psychol 1995; 104:41-54Crossref, Medline, Google Scholar
14. Rund BR: Backward-masking performance in chronic and nonchronic schizophrenics, affectively disturbed patients, and normal control subjects. J Abnorm Psychol 1993; 102:74-81Crossref, Medline, Google Scholar
15. Green MF, Nuechterlein KH, Breitmeyer B, Mintz J: Backward masking in unmedicated schizophrenic patients in psychotic remission: possible reflection of aberrant cortical oscillation. Am J Psychiatry 1999; 156:1367-1373Google Scholar
16. Schuck JR, Lee RG: Backward masking, information processing, and schizophrenia. Schizophr Bull 1989; 15:491-500Crossref, Medline, Google Scholar
17. Merritt RD, Balogh DW: Backward masking spatial frequency effects among hypothetically schizotypal individuals. Schizophr Bull 1989; 15:573-583Crossref, Medline, Google Scholar
18. Green MF, Nuechterlien KH, Mintz J: Backward masking in schizophrenia and mania, II: specifying the visual channels. Arch Gen Psychiatry 1994; 51:945-951Crossref, Medline, Google Scholar
19. Slaghuis WL, Curran CE: Spatial frequency masking in positive- and negative-symptom schizophrenia. J Abnorm Psychol 1999; 108:42-50Crossref, Medline, Google Scholar
20. Chen Y, Palafox GP, Nakayama K, Levy DL, Matthysse S, Holzman PS: Motion perception in schizophrenia. Arch Gen Psychiatry 1999; 56:149-154Crossref, Medline, Google Scholar
21. Chen Y, Levy DL, Nakayama K, Matthysse S, Palafox G, Holzman PS: Dependence of impaired eye tracking on deficient velocity discrimination in schizophrenia. Arch Gen Psychiatry 1999; 56:155-161Crossref, Medline, Google Scholar
22. Chen Y, Nakayama K, Levy DL, Matthysse S, Holzman PS: Psychophysical isolation of a motion-processing deficit in schizophrenics and their relatives and its association with impaired smooth pursuit. Proc Natl Acad Sci USA 1999; 96:4724-4729Google Scholar
23. O’Donnell BF, Swearer JM, Smith LT, Nestor PG, Shenton ME, McCarley RW: Selective deficits in visual perception and recognition in schizophrenia. Am J Psychiatry 1996; 153:687-692Link, Google Scholar
24. Stuve TA, Friedman L, Jesberger JA, Gilmore GC, Strauss ME, Meltzer HY: The relationship between smooth pursuit performance, motion perception, and sustained visual attention in patients with schizophrenia and normal controls. Psychol Med 1997; 27:143-152Crossref, Medline, Google Scholar
25. Levy DL, Holzman PS, Matthysse S, Mendell NR: Eye tracking and schizophrenia: a critical perspective. Schizophr Bull 1993; 19:461-536Crossref, Medline, Google Scholar
26. Slaghuis WL: Contrast sensitivity for stationary and drifting spatial frequency gratings in positive- and negative-symptom schizophrenia. J Abnorm Psychol 1998; 107:49-62Crossref, Medline, Google Scholar
27. Schwartz BD, McGinn T, Winstead DK: Disordered spatiotemporal processing in schizophrenics. Biol Psychiatry 1987; 22:688-698Crossref, Medline, Google Scholar
28. Merigan WH, Maunsell JHR: How parallel are the primate visual pathways? Annu Rev Neurosci 1993; 16:369-402Crossref, Medline, Google Scholar
29. Kaplan E: The receptive field structure of retinal ganglion cells in cat and monkey, in Vision and Visual Dysfunction. Edited by Leventhal AG. Boston, CRC Press, 1991, pp 10-40Google Scholar
30. Tootell RB, Hamilton SL, Switkes E: Functional anatomy of macaque striate cortex, IV: contrast and magno-parvo streams. J Neurosci 1988; 8:1594-1609Google Scholar
31. Van Sweden B, Van Erp MG, Mesotten F, Crevits L: Impaired early visual processing in disorganised schizophrenia. Acta Neurol Belg 1998; 98:17-20Medline, Google Scholar
32. Katsanis J, Iacono WG, Beiser M: Visual event-related potentials in first-episode psychotic patients and their relatives. Psychophysiology 1996; 33:207-217Crossref, Medline, Google Scholar
33. Schwartz BD, Nelson AV, Wall M, Winstead DK: Visual evoked potentials associated with neural filter mechanisms in schizophrenia. New Trends in Experimental and Clin Psychiatry 1985; 1:179-186Google Scholar
34. Jibiki I, Takizawa Y, Yamaguchi N: Visual dysfunction in treated schizophrenia suggested by visual evoked potentials from pattern-reversal. Eur Arch Psychiatry Clin Neurosci 1991; 241:61-64Crossref, Medline, Google Scholar
35. Schwartz BD, Winstead DK: Icon formation in chronic schizophrenics. Biol Psychiatry 1985; 20:1015-1018Google Scholar
36. Hardy LH, Rand G, Ritter MC: Tests for the detection and analysis of color blindness:1. the Ishihara test: an evaluation. J Opt Soc Am 1945; 35:268-275Google Scholar
37. Lizksz A: The Farnsworth Panel D-15 test. Am J Ophthalmol 1996; 62:27-37Crossref, Google Scholar
38. Lanthony P: The desaturated panel D-15. Doc Ophthalmol 1978; 46:185-189Medline, Google Scholar
39. Kay SR, Fiszbein A, Opler LA: The Positive and Negative Syndrome Scale (PANSS) for schizophrenia. Schizophr Bull 1987; 13:261-276Crossref, Medline, Google Scholar
40. Regan D: Human Brain Electrophysiology: Evoked Potentials and Evoked Magnetic Fields in Science and Medicine. New York, Elsevier, 1989Google Scholar
41. Tyler CW, Apkarian P, Levi DM, Nakayama K: Rapid assessment of visual function: an electronic sweep technique for the pattern visual evoked potential. Invest Ophthalmol Vis Sci 1979; 18:703-713Medline, Google Scholar
42. Norcia AM, Tyler CW: Spatial frequency sweep VEP: visual acuity during the first year of life. Vision Res 1985; 25:1399-1408Google Scholar
43. Zemon V, Hartmann EE, Gordon J, Prunte-Glowazki A: An electrophysiological technique for assessment of the development of spatial vision. Optom Vis Sci 1997; 74:708-716Crossref, Medline, Google Scholar
44. Zemon V, Gordon J, Welch J: Asymmetries in ON and OFF visual pathways of humans revealed using contrast-evoked cortical potentials. Vis Neurosci 1988; 1:145-150Crossref, Medline, Google Scholar
45. Zemon V, Gordon J: Spatial tuning characteristics of functional subsystems in the visual pathways of humans (abstract). Invest Ophthalmol Vis Sci Suppl 1988; 29:297Google Scholar
46. Zemon V, Siegfried J, Gordon J: Magno and parvo pathways in humans studied using VEPs to luminance and chromatic contrast (abstract). Invest Ophthalmol Vis Sci Suppl 1991; 32:1033Google Scholar
47. Greenstein VC, Seliger S, Zemon V, Ritch R: Visual evoked potential assessment of the effects of glaucoma on visual subsystems. Vision Res 1998; 38:1901-1911Google Scholar
48. Jasper HH: The ten-twenty electrode system of the International Federation. Electroencephalography J 1958; 10:371-375Google Scholar
49. Victor JD, Mast J: A new statistic for steady-state evoked potentials. Electroencephalogr Clin Neurophysiol 1991; 78:378-388Crossref, Medline, Google Scholar
50. Ungerleider LG, Mishkin M: Two cortical visual systems, in The Analysis of Visual Behavior. Edited by Ingle DJ, Mansfield RJW, Goodale MS. Cambridge, Mass, MIT Press, 1982, pp 549-586Google Scholar
51. Castet E, Masson GS: Motion perception during saccadic eye movements. Nat Neurosci 2000; 3:177-183Crossref, Medline, Google Scholar
52. Page WK, King WM, Merigan W, Maunsell J: Magnocellular or parvocellular lesions in the lateral geniculate nucleus of monkeys cause minor deficits of smooth pursuit eye movements. Vision Res 1994; 34:223-239Crossref, Medline, Google Scholar
53. Schroeder CE, Mehta AD, Giver SJ: A spatiotemporal profile of visual system activation revealed by current source density analysis in the awake macaque. Cereb Cortex 1998; 8:575-592Crossref, Medline, Google Scholar
54. Schwartz BD, Maron BA, Evans WJ, Winstead DK: High velocity transient visual processing deficits diminish ability of patients with schizophrenia to recognize objects. Neuropsychiatry Neuropsychol Behav Neurol 1999; 12:170-177Medline, Google Scholar
55. Bodis-Wollner I: Visual deficits related to dopamine deficiency in experimental animals and Parkinson’s disease patients. Trends Neurosci 1990; 13:296-307Crossref, Medline, Google Scholar
56. Jibiki I, Kurokawa K, Fukushima T, Yamaguchi N: Acutely administered haloperidol has little effect on steady-state visual evoked potentials from pattern-reversal stimulations in treated schizophrenics. Jpn J Psychiatry Neurol 1993; 47:51-55Medline, Google Scholar
57. Braff DL, Saccuzzo DP: Effects of antipsychotic medication on speed of information processing in schizophrenic patients. Am J Psychiatry 1982; 139:1127-1130Google Scholar