Changes in Levels of Phosphorus Metabolites in Temporal Lobes of Drug-Naive Schizophrenic Patients
Abstract
OBJECTIVE: The authors examined phospholipids and high-energy phosphorus metabolism in the temporal lobes of drug-naive schizophrenic patients. METHOD: In vivo 31P magnetic resonance spectroscopy was performed on 17 first-episode, drug-naive schizophrenic patients and 17 age- and gender-matched healthy subjects. RESULTS: Patients showed higher levels of phosphodiesters and lower levels of phosphomonoesters than the comparison group. Phosphocreatine levels were increased in the left temporal lobes of patients. CONCLUSIONS: The results suggest disturbed membrane phospholipid metabolism in both temporal lobes and decreased energy demands in the left temporal lobes of drug-naive schizophrenic patients.
In vivo 31P magnetic resonance spectroscopy (MRS) allows direct measurement of membrane phospholipids and high-energy phosphate metabolism in the brain (1). With 31P MRS, increased levels of phosphodiesters and decreased levels of phosphomonoesters have been observed in the frontal lobes of drug-naive schizophrenic patients (2, 3). Increased β-phosphates of ATP (β-ATP) and decreased inorganic orthophosphate also have been reported (2). When 31P MRS studies have included chronically medicated schizophrenic patients, findings have been inconsistent: while most investigators have found decreased phosphomonoesters or increased phosphodiesters or both (4–6), a recent study demonstrated a decrease in phosphodiester level in schizophrenic patients (7). These inconsistencies may be caused by differences in patient characteristics such as chronicity of illness, diagnostic subtype, and medication status, as well as the MRS method employed. 31P MRS specifically investigating the temporal lobes also has yielded contradictory results in medicated schizophrenic patients (5, 8, 9). Disturbed phospholipid metabolism has been proposed as a neurodevelopmental pathogenesis of schizophrenia (10). Under this hypothesis, MRS in the drug-naive state is likely to give more useful information for investigating pathophysiologic mechanisms of schizophrenia than studies of medicated patients. However, no report of MRS has described metabolite changes in the temporal lobes of drug-naive schizophrenic patients. In this study, we report metabolite changes observed in the temporal lobes of schizophrenic patients during initial psychotic episodes while they were still drug naive.
METHOD
Seventeen first-episode, drug-naive Japanese patients (10 men and seven women; mean age=23.1 years) who met DSM-III-R criteria for schizophrenia and were right-handed according to the Edinburgh Handedness Inventory were recruited from the outpatient clinics of Fujimoto Hospital and Kagoshima University Hospital from 1991 to 1996. One neuropsychiatrist evaluated the patients with the Oxford version of the Brief Psychiatric Rating Scale (BPRS). All the patients were treated in the two institutions, and their diagnosis was reconfirmed 1 year after the first scan. Patients had been ill for 6.6 months (SD=6.2). Seventeen age- and gender-matched healthy subjects (mean age=22.5 years) served as a comparison group. All subjects gave written informed consent for participation in the study. None had a recent history of alcohol or drug abuse.
The method of MRS data acquisition and processing has been described in our previous report (4). Spectroscopy was performed with a Siemens-Asahi Meditec MR system with a magnetic field strength of 2.0 T. A circular polarizing head coil was tuned to 84.5 MHz for proton imaging and to 34.2 MHz for in vivo multivoxel 31P MRS (two-dimensional chemical shift imaging). The field of view was 24 cm with an 8×8 data matrix and a 4-cm section thickness. Spectra were obtained from the two volumes of interest, each consisting of 72 ml (Figure 1). The TR was 2 seconds, and the TE was 1.72 msec. Twelve measurements were obtained for each spectrum. Data were processed with Fourier transformation and exponential multiplication (16 Hz) and then phase-corrected. Spectral peaks were obtained for phosphomonoesters, inorganic orthophosphate, phosphodiesters, phosphocreatine, and γ-, α-, and β-ATP. Spectra were quantified according to peak-area measurements. An automated baseline correction technique removed the distortion in the baseline of the spectra (11). Peak measures such as height, position, and width were obtained by a Lorentzian curve-fitting procedure (Figure 2). For each spectrum, the integrated areas of the seven metabolites were measured, and mole percentages of total phosphorus signal were calculated.
Repeated measures analysis of variance (ANOVA), with a between-subject factor of diagnosis and a within-subject factor of side, was applied to the mole percentage of the seven metabolites.
RESULTS
Significant effects of diagnosis were seen for phosphomonoesters and phosphodiesters, with increased levels of phosphodiesters and decreased levels of phosphomonoesters in both temporal lobes (Table 1). A significant diagnosis-by-side interaction was observed for phosphocreatine, with higher values on the left than on the right side in schizophrenic patients relative to healthy subjects. MR imaging revealed no obvious abnormalities in patients or healthy subjects. The mean BPRS score was 35.6 (SD=12.7). Kendall’s rank correlation coefficient revealed no significant correlation between the total BPRS score and percentages of phosphomonoesters (N=17; left: τ=0.17, p=0.34; right: τ=0.10, p=0.59), phosphodiesters (N=17; left: τ=0.04, p=0.84; right: τ=0.04, p=0.84), or phosphocreatine (N=17; left: τ=–0.07 p=0.68; right: τ=– 0.19, p=0.30).
DISCUSSION
In this study, 31P MRS detected an elevation of phosphodiesters and reduction of phosphomonoesters in the temporal lobes of drug-naive schizophrenic patients compared with healthy subjects. These results are consistent with previous observations reported in the prefrontal cortex of schizophrenic patients (2, 3). Pettegrew et al. (2) have speculated that decreased phosphomonoesters and increased phosphodiesters in the frontal lobes of schizophrenic patients may reflect decreased synthesis and increased breakdown of membrane phospholipids. However, the interpretation of the findings is not easy, as they previously suggested. Decreased phosphomonoesters resonance in this study may imply a reduction in freely mobile phosphomonoesters (phosphocholine, phosphoethanolamine) or less mobile molecules (including phosphorylated proteins) or both (1). Reduced synthesis of membrane phospholipids is one of these possibilities. The phosphodiester resonance in 31P MRS in vivo is believed to be derived from mobile phosphodiester moieties (small membrane phospholipid structures such as micelles and vesicles) and breakdown products (1, 12). Phosphodiesters are more concentrated in white than in the gray matter (13). Therefore, the increase in phosphodiesters could have resulted from increased mobile phosphodiester moieties including glycerophosphocholine and glycerophosphoethanolamine, as well as small membrane phospholipid structures, or it could have been a reflection of a decreased ratio of gray-to-white matter volume in the volume of interest (14). Which phosphodiester components contribute to the elevation of phosphodiester resonance could be distinguished by using 1H-decoupled 31P MRS. A preliminary study has shown that membrane or mobile phospholipids are increased in the frontal lobes of chronically medicated schizophrenic patients (6). On the other hand, elevations of glycerophosphocholine and glycerophosphoethanolamine concentrations have been demonstrated in the parietal lobes of young medicated schizophrenic patients compared with elderly schizophrenic patients and healthy subjects (15). Although definitive determination of the origins of decreased phosphomonoesters and increased phosphodiesters is difficult, the disturbed membrane phospholipid metabolism may not be restricted to the frontal lobe in the manner of the gray matter volume reduction observed in schizophrenic patients (16).
The level of phosphocreatine was increased in the left temporal lobe in schizophrenic patients. Phosphocreatine is known to be rapidly transformed to ATP when ATP is consumed by neuronal activity (17). An increased percentage of phosphocreatine may imply reduced ATP utilization in the left temporal lobe of drug-naive schizophrenic patients. This asymmetric abnormality in energy metabolism agrees with left-sided functional impairments observed in the temporal lobes of schizophrenic patients with single photon emission computed tomography (18).
Several methodologic limitations need to be addressed. The phosphorus metabolites were analyzed without correction for multiple comparison because of the small group size and the exploratory nature of this study, in spite of the increased risk of a type I error. Our curve-fitting method may have a drawback in that all seven metabolites were modeled as single spectral peaks, which could influence the results. Other methodologic limitations inherent in MRS procedures have been outlined in previous reports (8, 19). Further studies, in a larger group and with more sophisticated in vivo MRS techniques, will be needed to confirm our preliminary findings.
Received July 13, 1998; revision received Feb. 5, 1999; accepted Feb. 9, 1999. From the Department of Neuropsychiatry, Faculty of Medicine, Kagoshima University; and the South Japan Health Science Center, Miyazaki. Address reprint requests to Dr. Hiroshi Fukuzako, Department of Neuropsychiatry, Faculty of Medicine, Kagoshima University, 8-35-1 Sakuragaoka, Kagoshima 890-8520, Japan; [email protected] (e-mail) Supported by grants from the Ministry of Science, Culture, and Education (05770735 and 06770764) and the National Center of Psychiatry and Neurology of the Ministry of Health and Welfare (3A-5) of Japan (Dr. H. Fukuzako).
![]() |
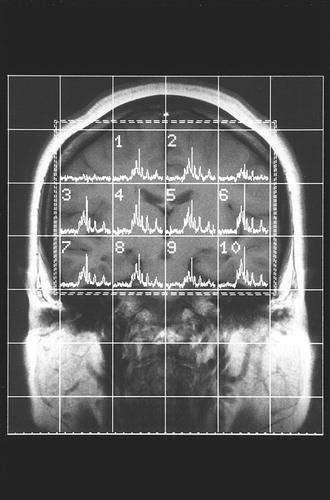
FIGURE 1. T1-Weighted MR Image Showing Placement of the Volume of Interest in a Patient With Schizophreniaa
aSpectra are obtained from the two volumes of interest (left: 6+10; right: 3+7).
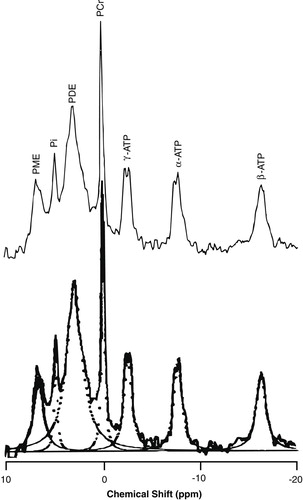
FIGURE 2. Typical in Vivo 31P MR Spectrum (upper panel) and Lorentzian Curve-Fitted Spectra (lower panel) for Each Metabolitea
aPME=phosphomonoesters, Pi=inorganic orthophosphate, PDE=phosphodiesters, PCr=phosphocreatine.
1. McClure RJ, Keshavan MS, Pettegrew JW: Chemical and physiologic brain imaging in schizophrenia. Psychiatr Clin North Am 1998; 21:93–122Crossref, Medline, Google Scholar
2. Pettegrew JW, Keshavan MS, Panchalingam K, Strychor S, Kaplan DB, Tretta MG, Allen M:Alterations in brain high-energy phosphate and membrane phospholipid metabolism in first-episode, drug-naive schizophrenics: a pilot study of the dorsal prefrontal cortex by in vivo phosphorus 31 nuclear magnetic resonance spectroscopy. Arch Gen Psychiatry 1991; 48:563–568Crossref, Medline, Google Scholar
3. Stanley JA, Williamson PC, Drost DJ, Carr TJ, Rylett RJ, Malla A, Thompson RT:An in vivo study of the prefrontal cortex of schizophrenic patients at different stages of illness via phosphorus magnetic resonance spectroscopy. Arch Gen Psychiatry 1995; 52:399–406Crossref, Medline, Google Scholar
4. Deicken RF, Calabrese G, Merrin EL, Meyerhoff DJ, Dillon WP, Weiner MW, Fein G: 31Phosphorus magnetic resonance spectroscopy of the frontal and parietal lobes in chronic schizophrenia. Biol Psychiatry 1994; 36:503–510Crossref, Medline, Google Scholar
5. Kegeles LS, Humaran TJ, Mann JJ: In vivo neurochemistry of the brain in schizophrenia as revealed by magnetic resonance spectroscopy. Biol Psychiatry 1998; 44:382–398Crossref, Medline, Google Scholar
6. Potwarka J, Drost DJ, Williamson PC: A study of schizophrenia using 2D 31P chemical shift imaging with 1H decoupling (abstract), in Proceedings of the 4th Scientific Meeting of the International Society for Magnetic Resonance in Medicine. New York, ISMRM, 1996, p 998Google Scholar
7. Volz H-P, Rzanny R, Rössger G, Hübner G, Kreitschmann-Andermahr I, Kaiser WA, Sauer H: 31Phosphorus magnetic resonance spectroscopy of the dorsolateral prefrontal region in schizophrenics—a study including 50 patients and 36 controls. Biol Psychiatry 1998; 44:399–404Crossref, Medline, Google Scholar
8. Deicken RF, Calabrese G, Merrin EL, Vinogradov S, Fein G, Weiner MW: Asymmetry of temporal lobe phosphorus metabolism in schizophrenia: a 31phosphorus magnetic resonance spectroscopic imaging study. Biol Psychiatry 1995; 38:279–286Crossref, Medline, Google Scholar
9. Fukuzako H, Fukuzako T, Takeuchi K, Ohbo Y, Ueyama K, Takigawa M, Fujimoto T: Phosphorus magnetic resonance spectroscopy in schizophrenia: correlation between membrane phospholipid metabolism in the temporal lobe and positive symptoms. Prog Neuropsychopharmacol Biol Psychiatry 1996; 20:629–640Crossref, Medline, Google Scholar
10. Horrobin DF: The membrane phospholipid hypothesis as a biochemical basis for the neurodevelopmental concept of schizophrenia. Schizophr Res 1998; 30:193–208Crossref, Medline, Google Scholar
11. Miyazaki T: Automatic baseline correction for 31P-MRS. Japanese J Magnetic Resonance in Medicine 1992; 12:232–237Google Scholar
12. McNamara R, Arias-Mendoza F, Brown TR: Investigation of broad resonances in 31P NMR spectra of the human brain in vivo. NMR Biomed 1994; 7:237–242Crossref, Medline, Google Scholar
13. Kilby PM, Allis JL, Radda GK: Spin-spin relaxation of the phosphodiester resonance in the 31P NMR spectrum of human brain: the determination of the concentrations of phosphodiester components. FEBS Lett 1990; 272:163–165Crossref, Medline, Google Scholar
14. Stanley JA, Panchalingam K, Miller G, McClure RJ, Pettegrew JW: A new method to quantify the broad component under the phosphodiester resonance and its application to study first-episode never medicated schizophrenics (abstract), in Proceedings of the 5th Scientific Meeting of the International Society for Magnetic Resonance in Medicine. Vancouver, ISMRM, 1997, p 1408Google Scholar
15. Blüml S, Tan J, Adatia N, Karme A, Sproull T, Harris K, Ross BD: Cerebral glycerophosphorylcholine and ethanolamine concentrations are elevated in young schizophrenics (abstract), in Proceedings of the 6th Scientific Meeting of the International Society for Magnetic Resonance in Medicine. Sydney, ISMRM, 1998, p 736Google Scholar
16. Lawrie SM, Abukmeil SS: Brain abnormality in schizophrenia: a systematic and quantitative review of volumetric magnetic resonance imaging studies. Br J Psychiatry 1998; 172:110–120Crossref, Medline, Google Scholar
17. Sappey-Marinier D, Calabrese G, Fein G, Hugg JW, Biggins C, Weiner MW: Effect of photic stimulation on human visual cortex lactate and phosphates using 1H and 31P magnetic resonance spectroscopy. J Cereb Blood Flow Metab 1992; 12:584–592Crossref, Medline, Google Scholar
18. Russell JM, Early TS, Patterson JC, Martin JL, Villanneva-Meyer J, McGee MD: Temporal lobe perfusion asymmetries in schizophrenia. J Nucl Med 1997; 38:607–612Medline, Google Scholar
19. Deicken RF, Calabrese G, Merrin EL, Fein G, Weiner MW: Basal ganglia phosphorous metabolism in chronic schizophrenia. Am J Psychiatry 1995; 152:126–129Link, Google Scholar