Lamina-Specific Alterations in the Dopamine Innervation of the Prefrontal Cortex in Schizophrenic Subjects
Abstract
OBJECTIVE: Abnormalities in dopamine neurotransmission in the prefrontal cortex have been implicated in the pathophysiology of schizophrenia. However, the integrity of the dopamine projections to the prefrontal cortex in this disorder has not been directly examined. METHOD: The authors employed immunocytochemical methods and antibodies against tyrosine hydroxylase, the rate-limiting enzyme in dopamine biosynthesis, and the dopamine membrane transporter to examine dopamine axons in the dorsomedial prefrontal cortex (area 9) from 16 pairs of schizophrenic and matched control subjects. RESULTS: Compared to the control subjects, the total length of tyrosine hydroxylase-immunoreactive axons was unchanged in the superficial and middle layers of the schizophrenic subjects but was reduced by an average of 33.6% in layer 6. The total length of tyrosine hydroxylase-positive axons in layer 6 was decreased in 13 of the schizophrenic subjects compared to their control subjects. Axons immunoreactive for the dopamine membrane transporter showed a similar pattern of change. In contrast, axons labeled for the serotonin transporter did not differ between schizophrenic and control subjects in any layer examined. In addition, the density of tyrosine hydroxylase-containing axons did not differ between monkeys chronically treated with haloperidol and matched control animals. CONCLUSIONS: These findings reveal that schizophrenia is associated with an altered dopamine innervation of prefrontal cortex area 9 that is lamina- and neurotransmitter-specific and that does not appear to be a consequence of pharmacological treatment. Together, these data provide direct evidence for a disturbance in dopamine neurotransmission in the prefrontal cortex of schizophrenic subjects.
In its original formulation, the dopamine hypothesis of schizophrenia posited that the psychotic symptoms of this disorder were due to a hyperdopaminergic state (1, 2). This hypothesis has undergone substantial revisions as a result of recent advances in our understanding of the complexity of dopamine systems and of the pathophysiology of schizophrenia. For example, perturbations of the dopamine system in schizophrenia have been suggested to be in opposite directions in different brain regions (3), such that a hyperdopaminergic state in subcortical structures coexists with a deficit in dopamine neurotransmission in the prefrontal cortex. The former has been proposed to account for the psychotic symptoms, and the latter has been proposed to contribute to the cognitive deficits and negative symptoms, that are characteristic of schizophrenia (4, 5).
The normal function of the prefrontal cortex clearly depends on an intact dopamine innervation (6–8), and several lines of evidence suggest that the dopamine innervation of the prefrontal cortex may be abnormal in schizophrenia. For example, schizophrenic subjects have been shown to have altered levels of expression of the mRNAs for some classes of dopamine receptors in the prefrontal cortex (9, 10). In addition, drug-naive schizophrenic subjects were reported to exhibit a decreased density of dopamine D1-like receptors in the prefrontal cortex, and the density of receptors was directly associated with performance on a cognitive task, the Wisconsin Card Sorting Test (11), that is dependent on the function of the prefrontal cortex. This notion of a hypodopaminergic state in the prefrontal cortex of schizophrenic subjects is also supported by the observation that dopamine agonists increase prefrontal cortex blood flow and that these metabolic changes are associated with improved performance on the Wisconsin Card Sorting Test (12, 13). Finally, chronic administration of phencyclidine, which mimics some symptoms of schizophrenia in humans, produces cognitive deficits and hypofunction of prefrontal cortex dopamine in monkeys (14). However, despite the substantial interest in the role of prefrontal cortex dopamine in the pathophysiology of schizophrenia, the integrity of dopamine afferents from the mesencephalon to the prefrontal cortex has not previously been examined in schizophrenic subjects.
We and others have previously described the dopamine innervation of the primate prefrontal cortex by using immunocytochemical methods and antibodies directed against dopamine, the dopamine membrane transporter, or tyrosine hydroxylase, the rate-limiting enzyme in catecholamine biosynthesis (15–19). In both monkeys and humans, dopamine afferents innervate all regions of the prefrontal cortex, with labeled axons being particularly dense in the dorsomedial region, area 9. In the present study, we used a similar approach to evaluate the relative density and laminar distribution of dopamine axons in postmortem specimens of area 9 from matched pairs of schizophrenic and normal control subjects.
METHOD
Human Tissue Specimens
Postmortem tissue specimens from 32 human brains were obtained during autopsies conducted at the Allegheny County Coroner’s Office. Written informed consent for brain donation and diagnostic interviews was obtained from the next of kin. These procedures were approved by the University of Pittsburgh’s Institutional Review Board for Biomedical Research.
Neuropathological examination revealed abnormalities in four subjects. In three (subjects 207, 213, and 313), occasional neuritic plaques were identified in the neocortex. However, the density of the plaques was insufficient to meet the diagnostic criteria for Alzheimer’s disease, and none of the subjects had a clinical history of dementia. The cause of death involved brain damage in two subjects (subject 207: subdural hematoma in the left parietal region; subject 517: vascular malformation and hemorrhage confined to the right temporal lobe), but no neuropathological abnormalities were detected in either subject in the region of interest for this study.
Consensus DSM-III-R diagnoses were made by an independent panel of experienced clinicians, who used information obtained from clinical records and structured interviews with one or more surviving relatives of each subject (20). Sixteen of the subjects had a diagnosis of schizophrenia or schizoaffective disorder (Table 1). One of the schizophrenic subjects (subject 234) had never been medicated; three (subjects 537, 621, and 207) had not taken neuroleptics for 10 months, 8 years, and 10 years, respectively, before death; and another subject (subject 185) was known to have been noncompliant with prescribed medications. The absence of medication in these subjects was confirmed by toxicology screens conducted on all subjects at the time of death. Two schizophrenic subjects (subjects 428 and 517) were being treated with the atypical antipsychotic agent clozapine at the time of death, and the remaining nine schizophrenic subjects were receiving typical neuroleptics.
As shown in Table 1, each schizophrenic subject was matched to one control subject for gender (the ratio of men to women was 10:6 in both groups) and race (with the exception of pair 16). Subjects were also matched as closely as possible for age and postmortem interval. The schizophrenic and control subjects did not differ in mean age (53.8 years, SD=9.4, and 54.6 years, SD=9.2, respectively) or postmortem interval (9.4 hours, SD=3.9, and 9.2 hours, SD=4.7, respectively). One control subject (subject 245) had a history of alcohol dependence. The remaining 15 control subjects had no known neurological, psychiatric, or substance abuse histories.
Tissue Preparation and Immunocytochemical Procedures
The left frontal lobe of each brain was cut into 1.0-cm-thick coronal blocks. Blocks were immersed in cold 4% paraformaldehyde in phosphate buffer for 48 hours and then stored in a cryoprotectant at –30°C (20). Mean tissue storage time in cryoprotectant did not significantly differ between schizophrenic (51.0 months, SD=21.9) and control (43.4 months, SD=28.9) subjects. In addition, previous studies have demonstrated that tissue storage under these conditions does not alter immunoreactivity for the antigens examined in this study (21). Blocks were sectioned coronally at 40 µm, and every 10th section was stained for Nissl substance with thionin. These sections were used to identify the location of area 9 on the superior frontal gyrus through use of the cytoarchitectonic criteria (20).
Floating tissue sections were processed for tyrosine hydroxylase or serotonin transporter immunoreactivity by using the avidin-biotin procedure and the Vectastain ABC Elite kit (Vector Laboratories, Burlingame, Calif.), as previously described (22), or for dopamine membrane transporter immunoreactivity by using a modification of the biotin amplification procedure (23). Tissue sections from each matched pair of schizophrenic and control subjects were always processed together. All slides were coded to conceal the subject number and diagnosis. The following antibodies were used in this study. 1) An affinity-purified sheep IgG1 antibody (supplied by Dr. J. Haycock, Louisiana State University, New Orleans), raised against tyrosine hydroxylase purified from rat pheochromocytoma (24), was used at a concentration of 0.7 µg/ml. 2) A rat antibody (Chemicon, Temecula, Calif.), raised against a fusion protein containing the N-terminus of the human dopamine membrane transporter protein (25), was used at a dilution of 1:2,000. 3) A rabbit antibody, raised against a fusion protein containing the carboxy-terminus of the human serotonin transporter (provided by Dr. Randy Blakely, Vanderbilt University, Nashville, Tenn.), was used at a dilution of 1:10,000. The specificity of each antibody has been demonstrated in immunoblot, immunoprecipitation, and immunocytochemical studies (19, 26–28).
Pharmacological Treatment and Tissue Preparation in Monkeys
To assess the possible influence of antipsychotic medications on the measures of interest in the human studies, we studied four male cynomolgus (Macaca fascicularis) monkeys, each of whom was treated chronically with the typical antipsychotic agent haloperidol decanoate, and four control animals matched for sex, age, and weight. Animals were stabilized with daily doses of haloperidol that produced 8-hour trough serum concentrations of 4–8 ng/ml, and then that dose was multiplied by 15 to obtain the dose of haloperidol decanoate to be administered intramuscularly every 4 weeks. The mean dose of haloperidol decanoate was 16.0 mg/kg (SD=2.1). Trough serum levels obtained just before the next dose averaged 4.3 ng/ml (SD=1.1); these levels have been shown to be associated with a therapeutic response in humans (29). Consistent with our attempt to mimic the clinical model of neuroleptic threshold dosing (30), all haloperidol-treated animals developed extrapyramidal symptoms that were effectively controlled with maintenance administration of benztropine mesylate. After 9–12 months of treatment, each animal was euthanized with an overdose of pentobarbital, and the brain was removed. Following a 45-minute postmortem interval, coronal tissue blocks from the left hemisphere were fixed by immersion in 4% paraformaldehyde and processed for tyrosine hydroxylase immunocytochemistry in a manner identical to that described earlier for the human subjects. All procedures were approved by the University of Pittsburgh’s Institutional Animal Care and Use Committee.
Qualitative and Quantitative Assessments of Tyrosine Hydroxylase-, Dopamine Membrane Transporter-, and Serotonin Transporter-Labeled Axons
Coded sections from matched pairs of human and monkey subjects were independently examined by three investigators (M.A., D.A.L., and J.N.P.) who were blind to subject number and group. Qualitative assessments of the relative density and laminar distribution of tyrosine hydroxylase-, dopamine membrane transporter-, and serotonin transporter-immunoreactive axons were rated for each subject, and differences, if evident, were used to rank order subjects within a pair. Assessments of three-way interrater agreement (Light’s kappa estimates [31]) for the four sets of comparisons were as follows: tyrosine hydroxylase-immunoreactive axons in humans, 0.88; dopamine membrane transporter-immunoreactive axons in humans, 0.83; serotonin transporter immunoreactive axons in humans, 0.79; and tyrosine hydroxylase-immunoreactive axons in monkeys, 1.0. For the small number of cases in which the raters’ independent assessments did not agree, slides were reviewed together in order to achieve consensus.
Quantitative studies were conducted by investigators (R.E.W., C.L.E., and C.M.) who were blind to subject number and group and who had not previously examined the tissue sections. Because the density of dopamine axons has previously been shown to be greater in medial than lateral area 9 (15, 16), quantitative studies were focused on the portion of area 9 located on the medial surface of the superior frontal gyrus. To obtain quantitative measures of axon length in the same laminar locations across brains, measurements of the distance from the pial surface to the borders of layers 1–2, 3–4, and 6–white matter were determined on the Nissl-stained sections from each brain. The ratios of the depth of each laminar border to the total cortical thickness were then used to identify the same borders in the adjacent sections processed for immunocytochemistry. In order to examine the laminar specificity of any changes in dopamine axons in schizophrenia, we sampled cortical layers 1, deep 3, and 6. These layers were selected for analysis because they contain the highest densities of dopamine axons in monkeys and humans (15–18) and because the substantial differences in the extrinsic connectivity of these three layers provide a means for interpreting the possible functional significance of any laminar-specific alterations in dopamine innervation.
Square sampling frames (10,000 µm2) were randomly placed in each of these three laminar locations on each tissue section with one axis of the sampling frame oriented parallel to the pial surface. Labeled processes were visualized under brightfield optics at a magnification of 600×, and the Eutectic Neuron Reconstruction System was used to reconstruct in three dimensions all labeled processes. The summed length of all reconstructed axons (total axon length) in each frame was determined and expressed as µm axon length/10,000 µm2. These procedures have been previously used to characterize differences in tyrosine hydroxylase- and dopamine membrane transporter-immunoreactive axons across experimental conditions in animals, and they have been externally validated by comparisons with biochemical measures in both developmental and lesion studies (21, 32, 33). Similar procedures were used in both human and monkey studies. The adequacy of the sampling procedures was assessed by calculations of the coefficient of error across sampling frames within each layer of each subject. The mean coefficient of error was 0.20 (SD=0.11) for the human studies and 0.07 (SD=0.04) for the monkey studies.
Statistical Analyses
For the human studies, the sample frames yielded three identifiable measurements in each of the three laminar locations on each tissue section. For the statistical analyses of axon length, the response variable was treated as a three-variate, multivariate observation of axon length. Within each laminar location, multivariate analysis of variance (MANOVA) was employed to analyze the multivariate axon length variable. The main effect in this MANOVA was the diagnosis variable (indicating whether the subject was schizophrenic or control), with blocking on the pairing of subjects and controlling for the covariates age, postmortem interval, and tissue storage time. The resulting F test for diagnosis compared schizophrenic and control subjects on the basis of the axon length measures, resulting in the F statistic having three degrees of freedom in the numerator. The same MANOVA model was used to assess the possible effects of the covariates beyond that controlled by pairing. Data summaries of axon length are based on the mean of the measures within each laminar location for each subject. For the monkey studies, paired t tests were used to assess differences between control and haloperidol-treated animals.
RESULTS
Tyrosine Hydroxylase- and Dopamine Membrane Transporter-Immunoreactive Axons in the Prefrontal Cortex of Schizophrenic and Control Subjects
Qualitative analyses conducted by investigators who were blind to diagnosis for each pair of subjects revealed that each control subject exhibited the regional and laminar patterns of distribution of tyrosine hydroxylase-immunoreactive axons characteristic of area 9 (15, 18), although the overall density of labeled axons differed across subjects. In 10 of the schizophrenic subjects, the density of tyrosine hydroxylase-immunoreactive axons was clearly reduced relative to that of their matched control subjects, and in some cases, this reduction was quite striking (Figure 1, parts A and B). In four of these schizophrenic subjects, the reduction in density of tyrosine hydroxylase-immunoreactive axons was present across all cortical layers, whereas in the other six subjects, the difference was evident only in the deep layers. Two of the schizophrenic subjects appeared to have an increase in tyrosine hydroxylase-immunoreactive axon density, and the remaining four subjects were judged to be similar to their matched control subjects.
Although the intensity of axon labeling was usually less robust with the dopamine membrane transporter antibody than with the tyrosine hydroxylase antibody in the same subject, the overall patterns of regional and laminar distribution of labeled axons were the same with both antibodies. Compared to control subjects, the density of dopamine membrane transporter-immunoreactive axons was rated as decreased in 10 schizophrenic subjects, increased in one, and unchanged in five. In some schizophrenic subjects, the decrease in density of dopamine membrane transporter-immunoreactive axons was marked, especially in the deep layers (Figure 2). With one exception, the difference in dopamine membrane transporter-immunoreactive axon density between a matched pair of subjects paralleled the difference in tyrosine hydroxylase-immunoreactive axon density.
Quantitative Analysis of Tyrosine Hydroxylase- Immunoreactive Axons in Schizophrenic and Control Subjects
To determine the magnitude of these differences, we measured the innervation density of tyrosine hydroxylase-immunoreactive axons because of the higher quality of labeling obtained with the anti-tyrosine hydroxylase antibody. The mean total length of tyrosine hydroxylase-immunoreactive axons in medial area 9 was decreased by 10.8% and 13.8% in layers 1 and 3, respectively, of the schizophrenic subjects compared to the control subjects (Figure 3). However, these differences in layers 1 and 3 did not achieve statistical significance (F=0.74 and 1.45, respectively, df=3, 10, p>0.30, MANOVA). In contrast, mean total axon length (per 10,000 µm2) in layer 6 of the schizophrenic subjects was significantly decreased, by 33.6%, compared to the matched control subjects (276.8 µm, SD=206.4, and 416.3 µm, SD=174.0, respectively) (F=5.23, df=3, 10, p=0.02, MANOVA). Comparison of individual matched pairs of subjects revealed that in 13 of the 16 schizophrenic subjects, the mean total axon length in layer 6 was less than that in the matched control subjects (Figure 4).
Serotonin Transporter-Immunoreactive Axons in Prefrontal Cortex of Schizophrenic and Control Subjects
In order to determine whether this reduction in the relative density of labeled axons in layer 6 of schizophrenic subjects was specific to dopamine axons or representative of a more generalized alteration in monoamine-containing afferents to the prefrontal cortex, we examined serotonin transporter-immunoreactive axons in the same 16 pairs of subjects. Both the relative density and laminar distribution of these fibers appeared unaltered in schizophrenic subjects. For example, qualitative evaluations did not reveal any apparent differences in serotonin transporter-immunoreactive axons between the same matched pair of schizophrenic and control subjects (Figure 1, parts C and D) that showed a marked reduction in tyrosine hydroxylase-immunoreactive axons (Figure 1, parts A and B). Quantitative studies confirmed that the total length of serotonin transporter-immunoreactive axons did not differ between the schizophrenic and control subjects in any layer (F=0.56, df=3, 10, p>0.40). In layer 6, the mean total length of serotonin transporter-immunoreactive axons for each schizophrenic subject was within the range of that of all control subjects (Figure 5, part B), whereas for eight of the schizophrenic subjects, mean total length of tyrosine hydroxylase-immunoreactive axons was lower than that of all control subjects (Figure 5, part A). It should also be noted that the total length of serotonin transporter-immunoreactive axons was less than that of tyrosine hydroxylase-immunoreactive axons in the control subjects (Figure 5). This finding is consistent with previous comparisons of the relative density of dopamine and serotonin axons in medial area 9 of the primate prefrontal cortex (34).
Assessment of Influence of Other Variables
Each schizophrenic subject was matched to a control subject to minimize the possible influence of gender, race, age, and postmortem interval on the measures of interest. When the pairing of subjects is taken into account, there were no significant effects of these variables, or of tissue storage time, on any measures of axon length. Correlational analyses also failed to detect any association between the magnitude of the decrease in tyrosine hydroxylase-immunoreactive axons in layer 6 of the schizophrenic subjects and age at onset or duration of illness (r<0.056, df=1, 14, p>0.84).
As shown in Figure 6, total length of tyrosine hydroxylase-immunoreactive axons in layers 1, 3, and 6 did not differ between monkeys treated chronically with the antipsychotic agent haloperidol and their matched control subjects.
DISCUSSION
The results of this study demonstrate a significant reduction in the relative density of axons immunoreactive for tyrosine hydroxylase and dopamine membrane transporter, two proteins that play a critical role in dopamine neurotransmission, in layer 6 of prefrontal cortex area 9 in subjects with schizophrenia. In contrast, cortical afferents immunoreactive for the serotonin transporter, a central protein in serotonin neurotransmission, did not differ between schizophrenic and control subjects. Furthermore, chronic treatment with haloperidol and benztropine did not alter the density of tyrosine hydroxylase-immunoreactive axons in the monkey prefrontal cortex; this suggests that our findings are not likely to be a consequence of the typical treatments used in schizophrenic subjects. Together, these findings provide evidence that the pathophysiology of schizophrenia includes a lamina- and neurotransmitter-specific alteration in the dopamine innervation of the prefrontal cortex.
Specificity of Alterations to Dopamine Innervation of the Prefrontal Cortex in Schizophrenia
The dopamine membrane transporter and tyrosine hydroxylase antibodies used in this study have both been shown to specifically label dopamine axons in the primate neocortex. The mRNA for the dopamine membrane transporter is expressed selectively in dopamine neurons, and the protein is confined to the cell bodies, dendrites, and axonal projections of these neurons (25). Although tyrosine hydroxylase is expressed in all catecholamine-containing neurons, multiple lines of evidence indicate that antibodies against tyrosine hydroxylase predominantly label dopamine axons in the primate neocortex (22). In both monkeys and humans, the distribution and morphology of cortical tyrosine hydroxylase-immunoreactive axons differ from those of axons immunolabeled for dopamine-beta-hydroxylase, a marker of noradrenergic structures (18, 35), and few cortical axons exhibit both tyrosine hydroxylase and dopamine-beta-hydroxylase immunoreactivity (36, 37). In contrast, direct comparisons of tyrosine hydroxylase-immunoreactive axons and those labeled with an anti-dopamine antibody revealed identical patterns of distribution in the monkey cortex (17, 37), and over 95% of all tyrosine hydroxylase-immunoreactive axons in the monkey prefrontal cortex are also dopamine membrane transporter-immunoreactive (19).
While our findings of a reduced density of tyrosine hydroxylase- and dopamine membrane transporter-immunoreactive axons are indicative of an alteration in dopamine afferents, they do not preclude the possibility of a concomitant reduction in other afferent systems to the prefrontal cortex in schizophrenia. Indeed, the magnitude of the reported decrease in synaptophysin (20, 38), a marker of axon terminals, in the prefrontal cortex of schizophrenic subjects cannot be accounted for solely by reductions in dopamine afferents. However, the absence of a difference between schizophrenic and control subjects in serotonin transporter-immunoreactive axons in the present study indicates that the observed change in dopamine axons does not reflect a nonspecific alteration in all monoaminergic projections to the prefrontal cortex in schizophrenia. These observations are also consistent with previous radioligand binding studies that found similar levels of serotonin uptake sites in the prefrontal cortex of schizophrenic and control subjects (39, 40).
Specificity of Alterations to the Disease Process of Schizophrenia
Several lines of evidence suggest that the findings of this study directly reflect the pathophysiology of the disease process and do not represent an epiphenomenon of its treatment or other factors. The absence of a change in serotonin transporter-immunoreactive axons in concert with the laminar-specific reduction in dopamine axons argues against the possibility that the present findings are a consequence of differences in the quality of the tissue between the two groups of subjects. In addition, previous investigations that included many of the subjects in the present study failed to find differences between the schizophrenic and control subjects in other immunocytochemical markers (41, 42). Most of the schizophrenic subjects included in the present study, but none of the control subjects, had been treated at some point in their lives with antipsychotic agents. However, the available evidence suggests that our findings are not the result of treatment with these agents, or of the anticholinergic drugs that are frequently used to treat the side effects of antipsychotic medications. First, our study included one schizophrenic subject who was never medicated and four subjects who were not receiving medications at the time of death. In each of these subjects, the density of tyrosine hydroxylase-immunoreactive axons in layer 6 was reduced compared to that in the matched control subjects (see pairs 1, 9, and 12–14 in Figure 4). Second, chronic treatment of monkeys with haloperidol and benztropine did not alter the relative density of tyrosine hydroxylase-immunoreactive axons in the prefrontal cortex. The absence of an effect of antipsychotic medications on the measures used in this study is supported by a recent report that chronic haloperidol administration in the rat did not affect tyrosine hydroxylase immunoreactivity in neurons of the mesocortical dopamine system, although it did decrease tyrosine hydroxylase immunoreactivity in the nigrostriatal system (43).
The possible influence of other clinical factors frequently associated with schizophrenia on tyrosine hydroxylase and dopamine membrane transporter immunoreactivity must also be considered. In the present study, six of the schizophrenic subjects had a history of alcohol abuse or dependence (Table 1). However, nine of the 10 schizophrenic subjects without any history of alcohol abuse or dependence also had a reduced density of tyrosine hydroxylase-immunoreactive axons in layer 6 compared to the matched control subjects (compare Table 1 and Figure 4). In addition, in one of the pairs of subjects (pair 4 in Table 1 and Figure 4), both the schizophrenic and the control subject had a history of alcohol dependence, and yet the density of tyrosine hydroxylase-immunoreactive fibers was still decreased in the schizophrenic subject.
Pathophysiological Significance
The finding of a relative decrease in innervation density of both tyrosine hydroxylase- and dopamine membrane transporter-immunoreactive axons in the prefrontal cortex of schizophrenic subjects suggests that either 1) the concentrations of both proteins are reduced in a subpopulation of dopamine axons, such that these axons are no longer detectable by immunocytochemical techniques, or 2) the dopamine axons containing these proteins are decreased in number. Although the first possibility cannot be excluded, we are not aware of any data that would directly support this interpretation. On the other hand, a reduced number of cortical dopamine axons might be expected to be associated with alterations in the dopamine neurons of the ventral tegmental area, which furnish many of the dopamine afferents to the prefrontal cortex. In the only study that has examined dopamine neurons in schizophrenic subjects, the number of neuromelanin-containing cells in the medial mesencephalon was reported to be decreased by 16%, although this difference was not significant (44). However, the somal volume of pigmented neurons was significantly reduced in the schizophrenic subjects. The latter findings could reflect a reduction in the size of the axon arbor of cortically projecting dopamine neurons, since somal size and total axon length tend to be positively correlated (45, 46).
Either reduced amounts of tyrosine hydroxylase and dopamine membrane transporter in dopamine axons or the presence of fewer dopamine axons could be associated with diminished dopamine neurotransmission in the prefrontal cortex of schizophrenic subjects. This possibility is consistent with the interpretation of other studies (see introduction) suggesting that schizophrenia is associated with a hypodopaminergic state in the prefrontal cortex. In addition, animal studies suggest that even relatively modest reductions in the density of dopamine axons in the prefrontal cortex can be associated with a substantial decrease in indices of dopamine neurotransmission. For example, in contrast to the nigrostriatal dopamine system in which residual dopamine terminals can compensate for the loss of the majority of axons in this projection (47), partial lesions of the dopamine mesocortical projection result in significantly decreased extracellular levels of dopamine in the prefrontal cortex (33). We do not know whether the magnitude of the decrease in prefrontal cortex dopamine axons observed in the present study of schizophrenia is sufficient to produce the types of cognitive impairments previously associated with reductions of prefrontal cortex dopamine in nonhuman primates (6–8). However, the similarities in cognitive deficits observed in these animal studies and in subjects with schizophrenia (48) suggest that the decrease in markers of prefrontal cortex dopamine axons observed in the present study could contribute to prefrontal cortex dysfunction in schizophrenia.
On the other hand, our findings of decreased tyrosine hydroxylase- and dopamine membrane transporter-immunoreactive axons do not necessarily provide evidence for a hypodopaminergic state in the prefrontal cortex of schizophrenic subjects. In fact, the complete absence of dopamine membrane transporter and reductions of 90% in tyrosine hydroxylase levels are associated with a markedly hyperdopaminergic state in the dopamine membrane transporter knockout mouse (49). Some studies have indicated that prefrontal cortex function may be impaired by either a deficiency or an excess of stimulation at prefrontal cortex dopamine D1 receptors (7, 50–52). Layer 6 of the primate prefrontal cortex contains a high density of dopamine D1-like receptors (53), and the density of these receptors has been reported to be decreased in drug-naive schizophrenic subjects (11). Since the symptoms of schizophrenia are frequently worsened by stress, which increases prefrontal cortex dopamine release (54), it is possible that decreases in pre- and postsynaptic dopamine markers in the prefrontal cortex represent a homeostatic response to minimize the impact of excessive levels of prefrontal cortex dopamine induced by environmental stress.
Although our findings reveal a lamina- and neurotransmitter-specific alteration in the dopamine innervation of the prefrontal cortex, other data suggest that these changes are likely to represent only one component of a more extensive set of alterations in prefrontal cortex circuitry in schizophrenia. For example, imaging and postmortem studies suggest that the mediodorsal thalamic nucleus, which furnishes the principal thalamic projection to the prefrontal cortex, may be reduced in size and contain fewer neurons in subjects with schizophrenia (55, 56). In addition, findings from other studies support the notion that the thalamic projections to the middle layers of the prefrontal cortex are decreased in schizophrenia (57, 58). Interestingly, the feedback projections from the prefrontal cortex to the mediodorsal thalamus originate from pyramidal neurons located in layers 5 and 6 (59), and the prefrontal cortex projections to the mediodorsal thalamus appear to play a prominent role in regulating thalamic activity (see reference 60 for a review). Since the dendritic shafts and spines of pyramidal cells are the principal synaptic target of dopamine axon terminals (61), and dopamine appears to play a critical role in regulating the influence of other inputs on pyramidal cell activity (62, 63), a shift in dopamine neurotransmission in prefrontal cortex layer 6 could reflect a change in the modulation of corticothalamic feedback in response to abnormal thalamocortical drive in schizophrenia.
Presented in part at the 22nd annual meeting of the Society for Neuroscience, Nov. 11–16, 1996. Received Nov. 24, 1998; revision received March 26, 1999; accepted April 12, 1999. From the Departments of Psychiatry, Neuroscience, and Statistics, University of Pittsburgh. Address reprint requests to Dr. Lewis, Western Psychiatric Institute and Clinic, University of Pittsburgh, Biomedical Science Tower, W1651, 3811 O’Hara St., Pittsburgh, PA 15213; [email protected] (e-mail). Supported by a Young Investigator Award from the National Alliance for Research on Schizophrenia and Depression (Dr. Akil) and by NIMH grants MH-00519, MH-45156, and MH-43784 (Dr. Lewis). The authors thank Drs. Gretchen Haas, Carol Sue Johnston, Matcheri Keshavan, and Nina Schooler for their participation in the diagnostic conferences; Ms. Sungyoung Auh and Ms. Katia Charland for statistical computations; and Ms. Mary Brady for photographic assistance.
![]() |
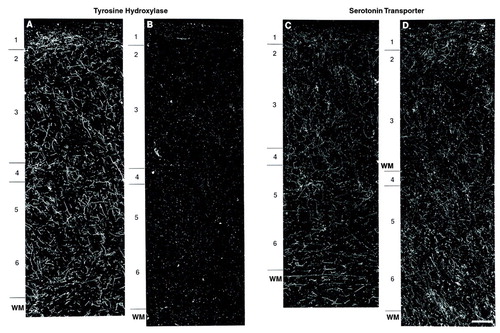
FIGURE 1. Dark-Field Photomicrographs of Tyrosine Hydroxylase-Immunoreactive (A, B) and Serotonin Transporter-Immunoreactive (C, D) Axons in Prefrontal Cortex Area 9 From a Schizophrenic (B, D) and Matched Control (A, C) Subject in Postmortem Studya
aPair 7; see table 1. Note the marked decrease in the density of tyrosine hydroxylase-immunoreactive axons across all cortical layers in the schizophrenic subject compared to the matched control subject, whereas the density of serotonin transporter-immunoreactive axons in adjacent sections does not appear to differ between subjects. Numerals indicate cortical layers, and WM indicates white matter. Calibration bar=200 µm and applies to all panels.
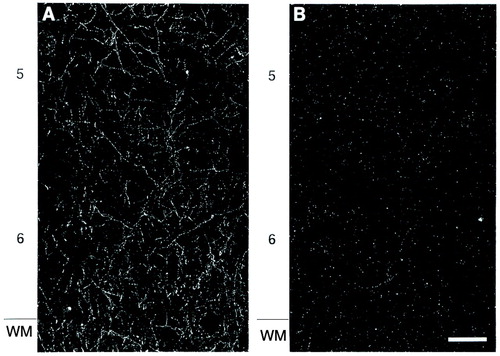
FIGURE 2. Dark-Field Photomicrographs of Dopamine Membrane Transporter-Immunoreactive Axons in Prefrontal Cortex Area 9 From a Control Subject (A) Matched to a Schizophrenic Subject (B) a
aPair 3; see table 1. Numerals indicate cortical layers, and WM indicates white matter. Calibration bar=200 µm.
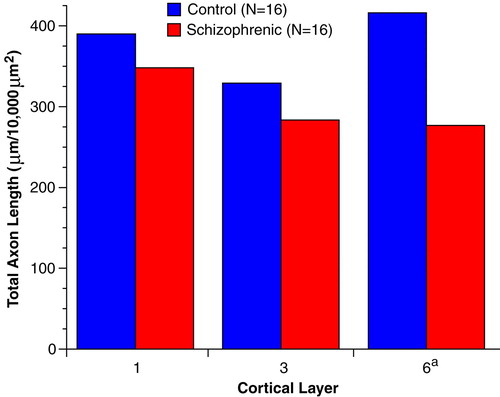
FIGURE 3. Mean Total Length of Tyrosine Hydroxylase-Immunoreactive Axons in Layers 1, 3, and 6 of Prefrontal Cortex Area 9 in Schizophrenic and Control Subjects in Postmortem Studya
aF=5.23, df=3, 10, p=0.02 (MANOVA).
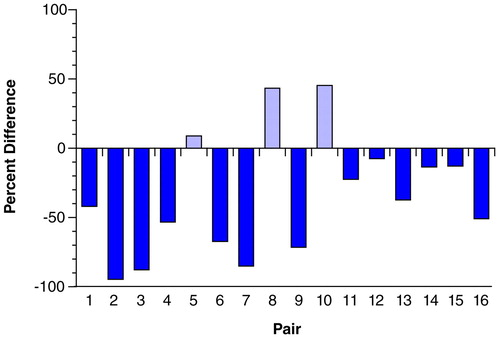
FIGURE 4. Percent Difference in Total Length of Tyrosine Hydroxylase-Immunoreactive Axons in Layer 6 of Prefrontal Cortex Area 9 for Each of 16 Matched Pairs of Schizophrenic and Control Subjectsa
aPair numbers are the same as those used in table 1. Note that the total length of tyrosine hydroxylase-immunoreactive axons is reduced in 13 of the schizophrenic subjects. Bars above 0 indicate pairs in which total axon length was increased in schizophrenic subjects, and bars below 0 indicate pairs in which total axon length was reduced in schizophrenic subjects relative to the matched control subjects.
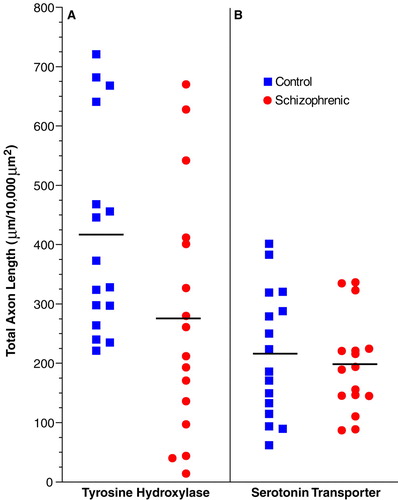
FIGURE 5. Mean Total Length of Tyrosine Hydroxylase-Immunoreactive (A) and Serotonin Transporter-Immunoreactive (B) Axons in Layer 6 of Prefrontal Cortex Area 9 in Schizophrenic and Control Subjectsa
aHorizontal lines indicate group means. Note that the total length of tyrosine hydroxylase-immunoreactive axons is decreased in the schizophrenic subjects compared to control subjects, whereas the total length of serotonin transporter-immunoreactive axons does not differ across groups.
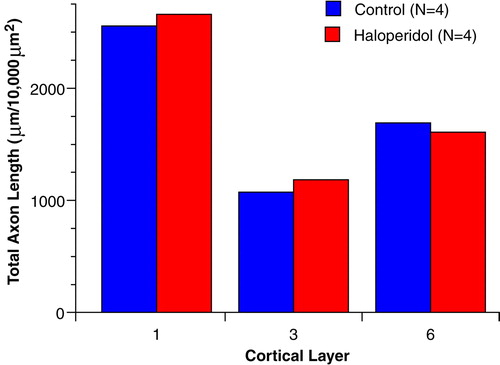
FIGURE 6. Mean Total Length of Tyrosine Hydroxylase-Immunoreactive Axons in Layers 1, 3, and 6 of the Prefrontal Cortex in Haloperidol-Treated and Matched Control Monkeysa
aGroup values did not differ in any layer (t<0.89, df=3, p>0.44, two-tailed test).
1. Snyder SH: Catecholamines in the brain as mediators of amphetamine psychosis. Arch Gen Psychiatry 1972; 27:169–179Crossref, Medline, Google Scholar
2. Carlsson A, Lindquist M: Effect of chlorpromazine and haloperidol on formation of 3-methoxytyramine and normetanephrine in mouse brain. Acta Pharmacol Toxicol 1963; 20:140–144Crossref, Medline, Google Scholar
3. Goldstein M, Deutch AY: Dopaminergic mechanisms in the pathogenesis of schizophrenia. FASEB J 1992; 6:2413–2421Google Scholar
4. Grace AA: Phasic versus tonic dopamine release and the modulation of dopamine system responsivity: a hypothesis for the etiology of schizophrenia. Neuroscience 1991; 41:1–24Crossref, Medline, Google Scholar
5. Davis KL, Kahn RS, Ko G, Davidson M: Dopamine in schizophrenia: a review and reconceptualization. Am J Psychiatry 1991; 148:1474–1486Google Scholar
6. Brozoski TJ, Brown RM, Rosvold HE, Goldman PS: Cognitive deficit caused by regional depletion of dopamine in prefrontal cortex of rhesus monkeys. Science 1979; 205:929–932Crossref, Medline, Google Scholar
7. Sawaguchi T, Goldman-Rakic PS: D1 dopamine receptors in prefrontal cortex: involvement in working memory. Science 1991; 251:947–950Crossref, Medline, Google Scholar
8. Arnsten AFT, Cai JX, Murphy BL, Goldman-Rakic PS: Dopamine D1 receptor mechanisms in the cognitive performance of young adult and aged monkeys. Psychopharmacology (Berl) 1994; 116:143–151Crossref, Medline, Google Scholar
9. Meador-Woodruff JH, Haroutunian V, Powchik P, Davidson M, Davis KL, Watson SJ: Dopamine receptor transcript expression in striatum and prefrontal occipital cortex. Arch Gen Psychiatry 1997; 54:1089–1095Google Scholar
10. Stefanis NC, Bresnik JN, Kerwin RW, Schofield WN, McAllister G: Elevation of D4 dopamine receptor mRNA in postmortem schizophrenic brain. Mol Brain Res 1998; 53:112–119Crossref, Medline, Google Scholar
11. Okubo Y, Suhara T, Suzuki K, Kobayashi K, Inoue O, Terasaki O, Someya Y, Sassa T, Sudo Y, Matsuchima E, Iyo M, Tateno Y, Toru M: Decreased prefrontal dopamine D1 receptors in schizophrenia revealed by PET. Nature 1997; 385:634–636Crossref, Medline, Google Scholar
12. Daniel DG, Weinberger DR, Jones DW, Zigun JR, Coppola R, Handel S, Bigelow LR, Goldberg TE, Berman KF, Kleinman JE: The effect of amphetamine on regional cerebral blood flow during cognitive activation in schizophrenia. J Neurosci 1991; 11:1907–1917Google Scholar
13. Daniel DG, Berman KF, Weinberger DR: The effect of apomorphine on regional cerebral blood flow in schizophrenia. J Neuropsychiatry 1989; 1:377–384Crossref, Medline, Google Scholar
14. Jentsch JD, Redmond DE Jr, Elsworth JD, Taylor JR, Youngren KD, Roth RH: Enduring cognitive deficits and cortical dopamine dysfunction in monkeys after long-term administration of phencyclidine. Science 1997; 277:953–955Crossref, Medline, Google Scholar
15. Lewis DA: The catecholaminergic innervation of primate prefrontal cortex. J Neural Transm 1992; 36:179–200Google Scholar
16. Lewis DA, Foote SL, Goldstein M, Morrison JH: The dopaminergic innervation of monkey prefrontal cortex: a tyrosine hydroxylase immunohistochemical study. Brain Res 1988; 449:225–243Crossref, Medline, Google Scholar
17. Williams SM, Goldman-Rakic PS: Characterization of the dopaminergic innervation of the primate frontal cortex using a dopamine-specific antibody. Cereb Cortex 1993; 3:199–222Crossref, Medline, Google Scholar
18. Gaspar P, Berger B, Fabvret A, Vigny A, Henry JP: Catecholamine innervation of the human cerebral cortex as revealed by comparative immunohistochemistry of tyrosine hydroxylase and dopamine-beta-hydroxylase. J Comp Neurol 1989; 279:249–271Crossref, Medline, Google Scholar
19. Lewis DA, Sesack SR, Levey AI, Rosenberg DR: Dopamine axons in primate prefrontal cortex: specificity of distribution, synaptic targets, and development, in Advances in Pharmacology. Edited by Goldstein D, Eisenhofer G, McCarty R. San Diego, Academic Press, 1998, pp 703–706Google Scholar
20. Glantz LA, Lewis DA: Reduction of synaptophysin immunoreactivity in the prefrontal cortex of subjects with schizophrenia: regional and diagnostic specificity. Arch Gen Psychiatry 1997; 54:943–952Crossref, Medline, Google Scholar
21. Erickson SL, Akil M, Levey AI, Lewis DA: Postnatal development of tyrosine hydroxylase- and dopamine transporter-immunoreactive axons in monkey rostral entorhinal cortex. Cereb Cortex 1998; 8:415–427Crossref, Medline, Google Scholar
22. Akil M, Lewis DA: The distribution of tyrosine hydroxylase-immunoreactive fibers in the human entorhinal cortex. Neuroscience 1994; 60:857–874Crossref, Medline, Google Scholar
23. Adams JC: Biotin amplification of biotin and horseradish peroxidase signals in histochemical stains. J Histochem Cytochem 1992; 40:1457–1463Google Scholar
24. Renfroe JB, Chronister RB, Haycock JW, Waymire JC: The localization of tyrosine hydroxylase-like immunoreactivity in the central nervous system: methodological considerations. Brain Res Bull 1984; 13:109–126Crossref, Medline, Google Scholar
25. Ciliax BJ, Heilman C, Demchyshyn LL, Pristupa ZB, Ince E, Hersch SM, Niznik HB, Levey AI: The dopamine transporter: immunochemical characterization and localization in brain. J Neurosci 1995; 15:1714–1723Google Scholar
26. Lewis DA, Melchitzky DS, Haycock JW: Four isoforms of tyrosine hydroxylase are expressed in human brain. Neuroscience 1993; 54:477–492Crossref, Medline, Google Scholar
27. Miller GW, Staley JK, Heilman CJ, Perez JT, Mash DC, Rye DB, Levey AI: Immunochemical analysis of dopamine transporter protein in Parkinson’s disease. Ann Neurol 1997; 41:530–539Crossref, Medline, Google Scholar
28. Qian Y, Melikian HE, Rye DB, Levey AI, Blakely RD: Identification and characterization of antidepressant-sensitive serotonin transporter proteins using site-specific antibodies. J Neurosci 1995; 15:1261–1274Google Scholar
29. Janicak PG, Davis JM, Preskorn SH, Ayd FJ: Principles and Practice of Psychopharmacotherapy. Baltimore, Williams & Wilkins, 1993Google Scholar
30. McEvoy JP, Schooler NR, Wilson WH: Predictors of therapeutic response to haloperidol in acute schizophrenia. Psychopharmacol Bull 1991; 27:97–101Medline, Google Scholar
31. Light RJ: Measures of response agreement for qualitative data: some generalizations and alternatives. Psychol Bull 1971; 76:356–377Crossref, Google Scholar
32. Rosenberg DR, Lewis DA: Postnatal maturation of the dopaminergic innervation of monkey prefrontal and motor cortices: a tyrosine hydroxylase immunohistochemical analysis. J Comp Neurol 1995; 358:383–400Crossref, Medline, Google Scholar
33. Venator DK, Lewis DA, Finlay JM: Effects of partial dopamine loss in the medial prefrontal cortex on local baseline and stress-evoked extracellular dopamine concentrations. Neuroscience (in press)Google Scholar
34. Lewis DA, Hayes TL, Lund JS, Oeth KM: Dopamine and the neural circuitry of primate prefrontal cortex: implications for schizophrenia research. Neuropsychopharmacology 1992; 6:127–134Medline, Google Scholar
35. Lewis DA, Campbell MJ, Foote SL, Goldstein M, Morrison JH: The distribution of tyrosine hydroxylase-immunoreactive fibers in primate neocortex is widespread but regionally specific. J Neurosci 1987; 7:279–290Crossref, Medline, Google Scholar
36. Noack HJ, Lewis DA: Antibodies directed against tyrosine hydroxylase differentially recognize noradrenergic axons in monkey neocortex. Brain Res 1989; 500:313–324Crossref, Medline, Google Scholar
37. Akil M, Lewis DA: The dopaminergic innervation of monkey entorhinal cortex. Cereb Cortex 1993; 3:533–550Crossref, Medline, Google Scholar
38. Perrone-Bizzozero NI, Sower AC, Bird ED, Benowitz LI, Ivins KJ, Neve RL: Levels of the growth-associated protein GAP-43 are selectively increased in association cortices in schizophrenia. Proc Natl Acad Sci USA 1996; 93:14182–14187Google Scholar
39. Dean B, Hayes W, Opeskin K, Naylor L, Pavey G, Hill C, Keks N, Copolov DL: Serotonin 2 receptors and the serotonin transporter in the schizophrenic brain. Behav Brain Res 1996; 73:169–175Crossref, Medline, Google Scholar
40. Gurevich EV, Joyce JN: Alterations in the cortical serotonergic system in schizophrenia: a postmortem study. Biol Psychiatry 1997; 42:529–545Crossref, Medline, Google Scholar
41. Woo T-U, Miller JL, Lewis DA: Schizophrenia and the parvalbumin-containing class of cortical local circuit neurons. Am J Psychiatry 1997; 154:1013–1015Google Scholar
42. Woo T-U, Whitehead RE, Melchitzky DS, Lewis DA: A subclass of prefrontal gamma-aminobutyric acid axon terminals are selectively altered in schizophrenia. Proc Natl Acad Sci USA 1998; 95:5341–5346Google Scholar
43. Levinson AJ, Garside S, Rosebush PI, Mazurek MF: Haloperidol induces persistent down-regulation of tyrosine-hydroxylase immunoreactivity in substantia nigra but not ventral tegmental area in the rat. Neuroscience 1998; 84:201–211Crossref, Medline, Google Scholar
44. Bogerts B, Hantsch J, Herzer M: A morphometric study of the dopamine-containing cell groups in the mesencephalon of normals, Parkinson patients, and schizophrenics. Biol Psychiatry 1983; 18:951–969Medline, Google Scholar
45. Lund JS, Lund RD, Hendrickson AE, Bunt AH, Fuchs AF: The origin of efferent pathways from the primary visual cortex, area 17, of the macaque monkey as shown by retrograde transport of horseradish peroxidase. J Comp Neurol 1975; 164:287–304Crossref, Medline, Google Scholar
46. Gilbert CD, Kelly JP: The projections of cells in different layers of the cat’s visual cortex. J Comp Neurol 1975; 63:81–106Crossref, Google Scholar
47. Snyder GL, Keller RW, Zigmond MJ: Dopamine efflux from striatal slices after intracerebral 6-hydroxydopamine: evidence for compensatory hyperactivity of residual terminals. J Pharmacol Exp Ther 1990; 253:867–876Medline, Google Scholar
48. Park S, Holzman PS: Schizophrenics show spatial working memory deficits. Arch Gen Psychiatry 1992; 49:975–982Crossref, Medline, Google Scholar
49. Giros B, Jaber M, Jones SR, Wightman RM, Caron MG: Hyperlocomotion and indifference to cocaine and amphetamine in mice lacking the dopamine transporter. Nature 1996; 379:606–612Crossref, Medline, Google Scholar
50. Murphy BL, Arnsten AFT, Goldman-Rakic PS, Roth RH: Increased dopamine turnover in the prefrontal cortex impairs spatial working memory performance in rats and monkeys. Proc Natl Acad Sci USA 1996; 93:1325–1329Google Scholar
51. Arnsten AFT, Goldman-Rakic PS: Noise stress impairs prefrontal cortical cognitive function in monkeys: evidence for a hyperdopaminergic mechanism. Arch Gen Psychiatry 1998; 55:362–369Crossref, Medline, Google Scholar
52. Williams GV, Goldman-Rakic PS: Modulation of memory fields by dopamine D1 receptors in prefrontal cortex. Nature 1995; 376:572–575Crossref, Medline, Google Scholar
53. Goldman-Rakic PS, Lidow MS, Gallagher DW: Overlap of dopaminergic, adrenergic, and serotoninergic receptors and complementarity of their subtypes in primate prefrontal cortex. J Neurosci 1990; 10:2125–2138Google Scholar
54. Thierry A-M, Tassin JP, Blanc G, Glowinski J: Selective activation of the mesocortical DA system by stress. Nature 1976; 263:242–244Crossref, Medline, Google Scholar
55. Andreasen NC, Arndt S, Swayze V II, Cizadlo T, Flaum M, O’Leary D, Ehrhardt JC, Yuh WTC: Thalamic abnormalities in schizophrenia visualized through magnetic resonance image averaging. Science 1994; 266:294–298Crossref, Medline, Google Scholar
56. Pakkenberg B: Pronounced reduction of total neuron number in mediodorsal thalamic nucleus and nucleus accumbens in schizophrenics. Arch Gen Psychiatry 1990; 47:1023–1028Google Scholar
57. Glantz LA, Lewis DA: Prefrontal cortical pyramidal neurons exhibit decreased dendritic spine density in subjects with schizophrenia. Arch Gen Psychiatry (in press)Google Scholar
58. Pierri JN, Edgar CL, Lewis DA: Somal size of prefrontal cortical pyramidal neurons in the thalamic recipient zone of subjects with schizophrenia. Abstracts of the Society for Neuroscience 1998; 24:987Google Scholar
59. Goldman-Rakic PS, Porrino LJ: The primate mediodorsal (MD) nucleus and its projection to the frontal lobe. J Comp Neurol 1985; 242:535–560Crossref, Medline, Google Scholar
60. Guillery RW, Feig SL, Lozs´di DA: Paying attention to the thalamic reticular nucleus. Trends Neurosci 1998; 21:28–32Crossref, Medline, Google Scholar
61. Goldman-Rakic PS, Leranth C, Williams SM, Mons N, Geffard M: Dopamine synaptic complex with pyramidal neurons in primate cerebral cortex. Proc Natl Acad Sci USA 1989; 86:9015–9019Google Scholar
62. Yang CR, Seamans JK: Dopamine D1 receptor actions in layers V–VI rat prefrontal cortex neurons in vitro: modulation of dendritic-somatic signal integration. J Neurosci 1996; 16:1922–1935Google Scholar
63. Penit-Soria J, Audinat E, Crépel F: Excitation of rat prefrontal cortical neurons by dopamine: an in vitro electrophysiological study. Brain Res 1987; 425:263–274Crossref, Medline, Google Scholar