SAD and the Not-So-Single Photoreceptors
Abstract
Research in the last century has demonstrated that light is a critical regulator of physiology in animals. More recent research has exposed the influence of light on human behavior, including the phenomenon of seasonal affective disorder (SAD). Repeated studies have shown that light treatment is effective in this disorder. The molecular mechanism by which the body absorbs the light that has energizing and antidepressant effects is still uncertain. This review presents evidence regarding the role of rod and cone photoreceptors, as well as the role of recently discovered nonvisual neuronal melanopsin-containing photoreceptors. The authors discuss an evolutionary-based theoretical model of humoral phototransduction. This model postulates that tetrapyrrole pigments, including hemoglobin and bilirubin, are blood-borne photoreceptors, regulating gasotransmitters such as carbon monoxide when exposed to light in the eye. Recent studies in an animal model for seasonality provide data consistent with this model. Understanding the molecular mechanisms by which light affects physiology may guide the development of therapies for SAD and other pathologies of circadian and circannual regulation.
For at least two millennia, physicians have associated winter with seasonal depression and summer with seasonal mania and have linked light to mood and energy (1). Early explorers of the northern polar regions noted their depression of mood and energy and the seasonal effects on human reproduction in native inhabitants associated with the severe darkness of winter in these latitudes. One U.S. naval medical officer noted in his 1854 diary that in the long Arctic winter night, “the influence of this long, intense darkness was most depressing” and that the return of the sun “was like bathing in perfumed water” (2–5).
Circannual rhythms of behavior are commonly observed among animals exposed to seasonal variations in temperature and daylight (6). Seasonal influences on the mood and behavior of humans, however, were largely ignored through most of the 20th century (7). In 1980 it was reported that bright light could suppress pineal gland melatonin production in humans (8), as earlier described in animals, and in 1984 winter seasonal depression (9), also known as seasonal affective disorder (SAD), was described. Winter SAD is characterized by seasonal experience of sadness, interpersonal difficulties, and physical symptoms including decreased activity, increased sleep time, carbohydrate craving, increased appetite, and weight gain. These symptoms usually respond to bright light therapy (9). A wide range of studies have demonstrated the efficacy of light treatment for SAD and have minimized the possibility that light treatment works by a placebo effect (10–12). Moreover, it has become clear that mild winter seasonal diminishment of mood and energy is common, even in people who do not qualify for a diagnosis of major depression (13). With these findings, the common link of light responsiveness between humans and animals could no longer be ignored.
A scientific mystery, however, was created. Researchers were left in the dark concerning the molecular mechanism by which humans absorb the light that has energizing and antidepressant effects. The First Law of Photochemistry, first articulated almost two centuries ago, postulates that light can produce a chemical reaction only by being absorbed by a molecule (14, 15). This fundamental law of nature means that for light to have a chemical effect, a molecule must directly mediate that effect. In the absence of knowing what molecule or molecules are being directly acted on by light, the physiology of light treatment of winter depression remains a black box.
In this review we will consider possible candidates for mediators of light effects in winter SAD, beginning with well-known light-absorbing molecules, and will consider novel evidence supporting more recently studied molecular mechanisms. Identification of the relevant photoreceptor or photoreceptors would truly open a window into the brain and the chronobiological physiology it regulates.
Photoreceptors in the Eye
Soon after the description and classification of SAD, a controlled trial compared the therapeutic effects of eye versus skin light treatment and provided evidence that light’s therapeutic effects in SAD were likely mediated through the eyes (16). These results were consistent with a wide body of literature establishing that light’s effects on the suprachiasmatic nuclei (the core mammalian biological clocks in the brain) are mediated through the eyes (17, 18).
Assuming that light’s effects were mediated through recognized photoreceptors, researchers initially hypothesized that the known visual photoreceptors, rod and/or cone rhodopsin-based molecules, were the primary receptors for circadian regulation and presumably for winter depression treatment as well (19–22).
The classical human photoreceptors are the rods and cones that permit vision through the eyes. Distributed in a layer throughout the back of the retina, rods and cones are precisely arrayed cells containing layers of various opsin molecules, which are specialized light absorbers that convert light energy into neural signals that are integrated in the brain to produce vision. Rod cells are sensitive to very dim light, permitting colorless vision in the night and near darkness. Cone cells are sensitive to brighter light, permitting color vision during the daytime and in bright light. Opsins are better able to absorb some specific wavelengths (or colors) of light than others: the three cone opsins in humans and primates absorb best in the red, green, and blue wavelengths of the spectrum. The integration of the neural signals from the different cones permits what humans sense as full color vision. As rod and cone opsins were the historically known photoreceptors in the eye, and as they had direct neural connections to the brain, it was logical to think that these also served as the primary mediators of light’s effects on the brain in winter SAD.
With a presumed ophthalmic pathway for light’s effects, it also became logical to consider that any disturbance predisposing to SAD could be found somewhere along the physiological pathways from eye to brain. Results of such investigations on the neurological front end of the visual system in SAD have been inconsistent, varying from no finding of significant visual processing abnormalities to depressive-state-related subsensitivities to environmental light (23–25). The molecular mechanism of visual process dysfunction in SAD has remained unclear, although recent work by Roecklein and colleagues (26) may be explanatory in a small percentage of people with the disorder (as discussed in the following section).
Using commonly accepted principles of photobiology, identification of putative photoreceptors is classically done by matching the wavelengths of light best able to have a desired effect with the wavelengths of light best absorbed by a candidate light-absorbing molecule (called a chromophore). If a molecule absorbs light best at the exact wavelengths at which the desired effect is seen, almost like a hand fitting in a glove, scientists consider this strong supportive evidence that the molecule under consideration is mediating the studied effect and is a relevant photoreceptor (27).
The first study that directly attempted to identify the photoreceptor(s) in SAD compared equal photon densities of blue versus red versus white light treatment for the disorder (28). Treatments were administered as white light containing all visible wavelengths (2,236 photopic lux), predominantly red light (603 lux), or predominantly blue light (638 lux). (Lux is a measure of how bright light appears to the human observer. Middle-wavelength or yellow to green photons appear brighter than long-wavelength red photons or short-wavelength blue photons.)
If stimulation of red or blue cone photoreceptor molecules mediating vision were mediating the antidepressant effects of light, the corresponding color of light should have proven effective. The failure of red or blue light to be effective in comparison to white light suggested to the researchers that the response in SAD was mediated by green-sensitive cones, rods (which also are maximally sensitive to green wavelengths), some combination of rods and cones, or a still unknown photoreceptor. Green light was then assumed to be likely to have antidepressant properties. A follow-up study compared green light (4,680 lux) and red light (603 lux). Photon densities were the same as those used in the prior study. Green light had a significantly better therapeutic effect than red light and appeared comparable in efficacy to the previously studied white light (29). These studies were consistent with the hypothesis that green light was effective, or most effective, in the treatment of SAD. In both of these studies, however, the brighter-appearing light, that is, the light with higher intensity in lux, was more effective, so these results are consistent with the alternative hypothesis that total apparent brightness (lux) determined therapeutic effect. In a third study of green light (2,367 lux) versus white light (1,103 lux), both proved therapeutic, and thus the results could not distinguish between these hypotheses. Together, these studies did suggest that the most effective wavelengths used in light treatment corresponded with those wavelengths that appeared brightest, i.e., were the best absorbed by the rods and cones for vision.
Recent data have shown that mice, which are nocturnally active, also have ultraviolet-sensitive (UV-sensitive) cones in the retina that play a major role in regulating their circadian physiology and sleep (30). Whether such cones exist in humans and whether they are applicable to SAD is still unknown. More broadly, the applicability of behavioral models based on nocturnally active rodents to diurnally active humans may have significant limits due to critical differences between species in the biology, physiology, and by definition, behavioral patterns and responses to light (31–33). Some controlled trials in SAD have explored whether UV light is necessary for treatment of SAD. These studies indicated that although UV wavelengths may or may not have antidepressant effects, UV light is not required for the treatment response and would therefore be contraindicated because of possible risks of stimulating skin cancers or ocular cataracts (29, 34–36).
Melanopsin
Much of the research into affective disorders and chronobiology grew out of a model linking advancement of the phase (timing) of the biological clock to antidepressant effects (37). Researchers made the assumption that the photoreceptors that mediate the antidepressant effects of light in SAD are the same as those that shift circadian rhythms in humans. As some evidence suggests that the antidepressant effect of light in SAD is effective in producing a phase advance (38, 39), the assumption is parsimonious and tenable. Circadian phase shifting has not been shown to be a requirement for light’s efficacy (39), however, and the assumption of identical photoreceptors for antidepressant and phase-shifting effects remains unproven.
Concurrent animal research further led to acknowledgment that rods and cones might not be the only critical photoreceptors for circadian rhythms (40). Following a report showing that hamster circadian rhythm photoreceptors exhibited properties that were unusual for rod and cone opsins (namely, the capacity for integration of time and intensity and a high threshold for bleaching) (41), serious consideration was given to the possibility that other molecules might mediate these effects. This view was supported in humans by the finding that exposing the eyes to 6,000 lux of white light could suppress melatonin production in some humans with complete visual blindness (42).
During the 1990s, work with mice with hereditary retinal degeneration, such as the rd/rd mouse, indicated that some novel photoreceptor must be involved in circadian entrainment. The rd/rd mice had no rods and few cones, had no perceptual sensitivity to light, but had fully preserved circadian entrainment (43). Some studies suggested the importance of a novel photoreceptive system with maximal sensitivity in the blue wavelengths; for instance, the maximum sensitivity for circadian phase shifting was near 480 nm in retinally degenerate mice, while the maximal sensitivity in normal mice was nearer 500 nm (44). Work in the transgenic rd/rd cl mouse, which has no cones or rods at all, eventually established that some novel non-rod, non-cone system must have the capability of driving the circadian rhythm system in rodents; presumably this would be true for humans also (45, 46).
Further research has demonstrated that the melanopsin system has a strong effect on the circadian system. This research has also shown the complexity of the effect of light on the brain. Knockout Opn4 −/− mice, which lack melanopsin, have altered circadian responses but are able to be entrained to light, implying that rods and cones do have access to the central clock as well (50, 51).
The discovery of melanopsin, whose absorption spectrum peaks in blue wavelengths, and subsequent work showing that blue (446–480 nm) wavelengths of light were particularly effective for suppression of melatonin production in humans (52, 53) led to renewed interest in blue light treatment for SAD. A number of other studies in humans have suggested that short-wavelength blue light is optimally effective for various circadian functions, including phase shifting (54, 55) and alertness induction (56). Two studies using light-emitting diodes studied the therapeutic effect of blue or blue-weighted light in SAD. In one study, narrow-band blue light-emitting diodes (398 lux) were convincingly more effective than dim narrow-band red light (23 lux) (57). A second study looked at the effect of 1,350-lux white light that was weighted toward the shorter-wavelength end of the spectrum, i.e., the energy distribution of the emitted light was characterized by having a main spectral emission peak at approximately 464 nm but also had a broader, secondary spectral peak near 564 nm (58). Of the energy emitted over the visible range of 400 to 700 nm, about 48% was emitted over the range 420 to 508 nm, and 37% was emitted over the range 512 to 616 nm. Collectively, the emitted light appeared bluish-white. Both studies obtained therapeutic effects similar to those obtained in studies using typical 10,000-lux broadband white light. A separate recent study showed no difference between “blue-enriched” and white light and found no superiority of blue over white light, although both conditions may have produced a maximal treatment response (59). Human data suggesting that green-wavelength light (approximately 500 nm) can be highly effective in shifting rhythms and in suppressing melatonin emphasizes that middle-wavelength light does access the circadian rhythm system (60). As already noted, green light does appear to have a potential therapeutic effect in SAD, whether through stimulation of the melanopsin system or through classical rod and cone photoreceptors. At this time, the most effective wavelengths of light for treatment of SAD remain unclear.
Melanopsin researchers then began to consider specifically whether melanopsin might play a role in mediating light’s effects in SAD. Hypothesizing that variations in melanopsin candidate genes might affect light input to the brain and increase vulnerability to SAD, one study explored haplotypes of the melanopsin gene, including specific single nucleotide polymorphisms that result in coding variants of the melanopsin protein (61). A single missense variant (P10L) was associated with increased risk of SAD, specifically for individuals homozygous for the minor T allele when compared to other genotypes (C/T and C/C). This variant was seen in 5% of individuals with SAD. Further work by the Pittsburgh research team has indicated a modest mean decrease in the post blue light illumination pupillary reflex regulated by melanopsin in a small group with SAD (26). It would be important to discover how light treatment mediates the antidepressant and energizing effect of light in these people.
Humoral Phototransduction
But what of the 95% of people with SAD for whom an abnormality in melanopsin phototransduction does not explain their vulnerability to seasonal change? Do most SAD patients have normal melanopsin-mediated light absorption but abnormal processing of the melanopsin-mediated light signal beyond the melanopsin molecule? Might there be a different photoreceptor that mediates seasonal or antidepressant effects of light in addition to the circadian effects of light that are mediated by melanopsin, and to some degree rods and cones?
While Darwin reported in 1880 that “hardly anyone supposes that there is any real analogy between the sleep of animals and that of plants” (62), seasonal and circadian behaviors of plants have been observed for millennia.
Drawing specifically on this evolutionary argument that seasonal and circadian influences of light have significant behavioral similarities in plants and animals, a model of “humoral phototransduction” was proposed (63). This model argued that the well-known tetrapyrrole-based light-absorbing molecules that regulate plant energy and chronobiology might have analogues in animal biology and, in particular, proposed that blood-borne tetrapyrroles directly mediate light’s effects in SAD through the eyes. In plants these molecules are chlorophyll, containing a closed-ring tetrapyrrole, and phytochrome, an open-ring tetrapyrrole. Synthesized along identical molecular structural pathways in plant and animal species until the final chemical reactions that make them distinct, the light-absorbing chromophore structures of chlorophyll and phytochrome are analogous to the light-absorbing chromophores of hemoglobin and its breakdown products of biliverdin and bilirubin. As the retina is highly vascular with blood vessels unshielded by protecting skin or tissue, the eye is the site of the most efficient exposure of blood to light. In this model, the pigments mediating the effects of light include the plant chlorophyll analogue hemoglobin, the phytochrome analogues biliverdin and bilirubin, and hemoproteins, such as heme oxygenase and nitric oxide synthase.
A novel and direct prediction of this “humoral phototransduction” model is that light will stimulate the release by hemoglobin and production by hemoproteins of what today are described as the “gasotransmitters” carbon monoxide (CO) and nitric oxide (NO) in retinal venous blood. These messengers could then drain to the cavernous venous sinus plexus. The cavernous venous sinus plexus has long been considered a remarkable structure of multiple blood vessels enwrapping the internal carotid artery (Figure 1). These gaseous transmitters could then diffuse into the internal carotid artery and provide a humoral signal of daylight to the adjacent suprachiasmatic nuclei. Countercurrent mechanisms for local chemical regulation have been demonstrated in diverse fish and animal physiological systems for decades. A countercurrent transfer of reproductive hormones from venous to arterial blood has indeed been demonstrated in the cavernous sinus (64).

a Illustration by Jeanette Kuvin Oren; adapted from reference 63.
A key building block of this model was recently confirmed by Koziorowski and colleagues, who examined seasonal and diurnal variation in venous blood CO from the eye in a male wild boar-pig crossbreed (65). This animal model is particularly useful for study both because of the large seasonal variation in the animal’s behavior and because of the accessibility of its relatively large major eye and brain blood vessels, which are generally similar in structure to those of humans. Koziorowski et al. compared CO levels in ophthalmic and nasal venous blood in the morning, afternoon, and night in eight animals during the longest days of the year and eight animals during the shortest days of the year at 50°N latitude.

a Adapted from reference 65; reprinted by permission of Biolife S.A.S.
b Morning and afternoon ophthalmic venous CO concentrations both significantly higher than the nasal venous CO concentrations and higher than the nocturnal ophthalmic venous concentration (N=8, p<0.05).
c Daytime and nocturnal ophthalmic venous CO concentrations both significantly higher than the nasal venous concentrations (N=8, p<0.05).
There are at least two plausible (and complementary) mechanisms for the observation of elevated CO seen in retinal venous blood after bright summer light. First would be the photodissociation of CO bound to heme iron groups in a reaction that was discovered in the 19th century by Haldane and Lorrain Smith (66). This process was dismissed as clinically irrelevant by its discoverers and long ignored by biologists owing to the mistaken belief that CO was biologically inert, except as a poison that interfered with hemoglobin’s normal oxygen-carrying capacity. A second mechanism would be the well-documented stimulation by bright light of heme oxygenase, the enzyme that cleaves heme moieties to form CO and biliverdin (67). This photostimulation simultaneously dissociates bound CO from the heme moiety of heme oxygenase and activates the enzyme itself to produce more CO.
Biliverdin is immediately converted in mammals to bilirubin by biliverdin reductase. Along with melatonin (68), biliverdin and bilirubin are among nature’s most potent antioxidants (69). Bilirubin is a photoactive molecule with daily and seasonal variation, rising at night and declining in the day, higher during the months when the days shorten and lower during the months when the days lengthen (70, 71). Bilirubin’s daily and seasonal links have been less well studied than those of melatonin. Bilirubin thereby parallels melatonin in having circadian and circannual rhythms, antioxidant properties, and regulation by light. It might also play an important role in regulating seasonal rhythms. Qualitatively, it exhibits a circadian signal that is different from that of melatonin. Whereas peripheral melatonin levels regularly begin to rise around dusk, peak in the middle of the night, and decline to baseline by dawn (72), peripheral bilirubin levels climb during the night and peak near dawn, then decline during the daylight to reach their minima near dusk (70). In contrast to melatonin, bilirubin might provide a distinct and complementary humoral signal of environmental light and internal time to the body.
Preliminary data supporting a link between bilirubin and SAD was subsequently found in a study in which nocturnal bilirubin levels in patients with winter depression were shown to be significantly lower than those in age- and gender-matched normal comparison volunteers and were significantly higher after morning light treatment, commensurate with clinical improvement (73).
Furthermore, bilirubin is a tetrapyrrole and is itself sensitive to light. The sensitivity of hyperbilirubinemia to bright light has been known to be of therapeutic importance for neonatal jaundice for decades (74). In the presence of sufficiently bright light, the normal 4Z,15Z-bilirubin form quickly undergoes reversible configural isomerization to form 4Z,15E-bilirubin (see Figure 3). The optimal wavelengths for stimulating this reaction lie in the blue region of the spectrum (74). The latter isomer is a less lipophilic form of bilirubin that is more easily excreted unchanged in bile. Bright light also can rapidly and irreversibly convert normal 4Z,15Z-bilirubin to another structural isomer: Z-lumirubin. This form is also less lipophilic than its parent bilirubin compound and can also be excreted in urine, unlike normal bilirubin, which is normally excreted only in bile (74, 76). As such, both 4Z,15E-bilirubin and Z-lumirubin would also be less likely to have the capacity to diffuse from the cavernous sinus into the internal carotid artery and signal dark to the brain. In a pilot study, whose results correspond with this proposal, during light therapy in adults, within 10 minutes statistically significant increases in lumirubin levels were seen in urine, and within 50 minutes statistically significant decreases in bilirubin were seen in plasma (77). Bilirubin is an attractive photoreceptor candidate for mediating some of light’s effects in SAD and in affecting circadian chronobiological rhythms. Bilirubin might therefore be both a signal and a transducer.
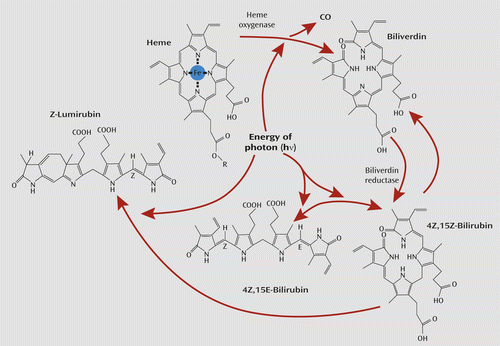
a Bilirubin’s activity as an antioxidant is demonstrated by its own oxidation back to biliverdin (75).
In short, the tetrapyrrole pigments of blood are photoactive and are photoactive at environmental levels of light exposure. Exposure of the eye to light appears to cause a physiological release of CO and may generate biliverdin, which is rapidly converted to 4Z,15Z-bilirubin. This form of bilirubin itself is converted by light to two less lipophilic isomers. Our model thus generates multiple signals of environmental light. Only empirical data will reveal the nature of the postulated tetrapyrrole signal to the brain. On the basis of the preceding data, it seems likely that retinal venous CO is a potential biomarker of circadian or seasonal rhythms and is directly affected by light’s actions on retinal blood. Nevertheless, these data by themselves do not prove that retinal venous CO or other gasotransmitters such as NO, or bilirubin, are in fact providing specific signals to the brain and regulating circadian or seasonal responses. Demonstration of changes in levels of chronobiological biomarkers regulated by the suprachiasmatic nuclei (such as peripheral melatonin levels) by the infusion of exogenous CO or NO in ophthalmic venous blood and demonstration of countercurrent transfer of CO or NO across the cavernous sinus into the internal carotid artery or increased delivery of CO or NO to the suprachiasmatic nuclei would provide direct evidence of a humoral pathway from eye to brain. Such studies are underway.
Challenges and Conclusion
The primary photoreceptors that mediate light’s seasonal effects and its antidepressant effects in SAD remain unclear: the candidates include melanopsin, the rod and cone opsins (either through their direct connection to the brain or through their input to the melanopsin system), and the tetrapyrroles of hemoproteins, biliverdin, and bilirubin as discussed here. The panoply of data suggests that light’s varied effects may be mediated through a small constellation of chromophores.
This new perspective recognizes what science has been demonstrating for most of the past century, that light is a core regulator of animal biology, as commonly accepted in plants. Such a view is tenable in the context of the conservation of molecular structure of the tetrapyrrole chromophores and their biosynthetic pathways in plants and animals (63). This view is supported by later discoveries, many of which are still emerging, of significant commonalities of plant and animal physiology, including circadian regulation, seasonal regulation, and sensory regulation (78). If hemoproteins and bilirubin are physiological photosensors, then the ubiquitous presence of hemoglobin and its breakdown products at the core of animal biology surely places light and photosensitive regulation of bioactive gases at the core of animal physiology as well. This new perspective echoes the wisdom of the ancients of millennia ago, who recognized light’s physiological benefits for human energy and mood (79). For that matter, the ancients may have been correct in some respects as well in linking bile and mood.
Understanding the mechanism of light’s therapeutic effect in SAD is important not only theoretically but practically. Knowing the most effective wavelength distribution may enable more efficient and more effective treatment devices. Even if a particular wavelength is found most therapeutically potent, it may not be the optimal clinical approach. For example, short-wave blue light is potentially phototoxic to the retina, and light of longer wavelength could prove safer (80). In terms of SAD, the research agenda suggested by the First Law of Photochemistry once called simply for matching a single color of light causing a clinical response with a single color of light that a pigment absorbs. The research agenda for understanding light’s effects on human physiology today is certainly less simple but, in photochemical language, most exciting.
1 : A History of Ancient Psychiatry. Westport, Conn, Greenwood Press, 1986Google Scholar
2 : Arctic Explorations in the Years 1853, ′54, ′55. Philadelphia, Childs and Peterson, 1856Google Scholar
3 : Gynecology and obstetrics among the Eskimos. Brooklyn Med J 1894; 8:154–169Google Scholar
4 : Medical observations among the Esquimaux. NY J Gyn Ob. 1894; 4:282–286Google Scholar
5 : Through the First Antarctic Night. New York, Doubleday & McClure, 1900Google Scholar
6 : Circannual systems, in Handbook of Behavioral Neurobiology. Edited by Aschoff J. New York, Plenum, 1981, pp 381–389Crossref, Google Scholar
7 : Seasonal affective disorders: a historical overview, in Seasonal Affective Disorders and Phototherapy. Edited by Rosenthal NEBlehar MC. New York, Guilford, 1989, pp 11–32Google Scholar
8 : Light suppresses melatonin secretion in humans. Science 1980; 210:1267–1269Crossref, Medline, Google Scholar
9 : Seasonal affective disorder: a description of the syndrome and preliminary findings with light therapy. Arch Gen Psychiatry 1984; 41:72–80Crossref, Medline, Google Scholar
10 : Light therapy for seasonal affective disorder: a review of efficacy. Neuropsychopharmacology 1989; 2:1–22Crossref, Medline, Google Scholar
11 : Dawn and dusk simulation as a therapeutic intervention. Biol Psychiatry 1989; 25:966–970Crossref, Medline, Google Scholar
12 : Light therapy for winter depression, in Biologic Effects of Light. Edited by Holick MKligman AM. Berlin, De Gruyter, 1992, pp 171–187Crossref, Google Scholar
13 : Phototherapy in individuals with and without subsyndromal seasonal affective disorder. Arch Gen Psychiatry 1989; 46:837–844Crossref, Medline, Google Scholar
14 : John William Draper, in Dictionary of Scientific Biography. Edited by Gillispie CC. New York, Scribner's, 1971, pp 181–183Google Scholar
15 : Theodor (Christian Johann Dietrich) von Grotthuss, in Dictionary of Scientific Biography. Edited by Gillispie CC. New York, Scribner's, 1971, pp 558–559Google Scholar
16 : Eye versus skin phototherapy of seasonal affective disorder. Am J Psychiatry 1987; 144:753–757Link, Google Scholar
17 : Neural mechanisms for entrainment and generation of mammalian circadian rhythms. Fed Proc 1979; 38:2589–2595Medline, Google Scholar
18 : Twilight times: light and the circadian system. Photochem Photobiol 1997; 66:549–561Crossref, Medline, Google Scholar
19 : Effect of light wavelength on the suppression of nocturnal plasma melatonin in normal volunteers. Ann N Y Acad Sci 1985; 453:376–378Crossref, Google Scholar
20 : Action spectrum of the retinal mechanism mediating nocturnal light-induced suppression of rat pineal gland N-acetyltransferase. Brain Res 1987; 406:352–356Crossref, Medline, Google Scholar
21 : The suppression of nocturnal pineal melatonin in the Syrian hamster: dose-response curves at 500 and 360 nm. Endocrinology 1987; 121:266–270Crossref, Medline, Google Scholar
22 : Action spectra of the lateral eyes recorded from mammalian pineal glands. Brain Res 1987; 424:10–16Crossref, Medline, Google Scholar
23 : An investigation of ophthalmic function in winter seasonal affective disorder. Depression 1993; 1:29–37Crossref, Google Scholar
24 : Effects of season on electro-oculographic ratio in winter seasonal affective disorder. Psychiatry Res 1995; 59:151–155Crossref, Medline, Google Scholar
25 : Evidence of a biological effect of light therapy on the retina of patients with seasonal affective disorder. Biol Psychiatry 2009; 66:253–258Crossref, Medline, Google Scholar
26 : The post illumination pupil response is reduced in seasonal affective disorder. Psychiatry Res (Epub ahead of print June 28, 2013)Google Scholar
27 : Life Processes of Plants. New York, Scientific American Library, 1994Google Scholar
28 : Effects of different wavelengths in seasonal affective disorder. J Affect Disord 1990; 20:209–216Crossref, Medline, Google Scholar
29 : Treatment of seasonal affective disorder with green light and red light. Am J Psychiatry 1991; 148:509–511Link, Google Scholar
30 : Ultraviolet light provides a major input to non-image-forming light detection in mice. Curr Biol 2012; 22:1397–1402Crossref, Medline, Google Scholar
31 : Retinas of the diurnal rodent Arvicanthis ansorgei are highly resistant to experimentally induced stress and degeneration. Invest Ophthalmol Vis Sci 2011; 52:8686–8700Crossref, Medline, Google Scholar
32 : Lab mice in the field: unorthodox daily activity and effects of a dysfunctional circadian clock allele. J Biol Rhythms 2011; 26:118–129Crossref, Medline, Google Scholar
33 : Circadian rhythms and depression: human psychopathology and animal models. Neuropharmacology 2012; 62:101–114Crossref, Medline, Google Scholar
34 : Exposure to ultraviolet B radiation during phototherapy. Am J Psychiatry 1990; 147:675–676Link, Google Scholar
35 : The effects of ultraviolet-A wavelengths in light therapy for seasonal depression. J Affect Disord 1992; 24:237–243Crossref, Medline, Google Scholar
36 : Ultraviolet versus non-ultraviolet light therapy for seasonal affective disorder. J Clin Psychiatry 1991; 52:213–216Medline, Google Scholar
37 : Phase advance of the circadian sleep-wake cycle as an antidepressant. Science 1979; 206:710–713Crossref, Medline, Google Scholar
38 : Morning vs evening light treatment of patients with winter depression. Arch Gen Psychiatry 1998; 55:890–896Crossref, Medline, Google Scholar
39 : Circadian time of morning light administration and therapeutic response in winter depression. Arch Gen Psychiatry 2001; 58:69–75Crossref, Medline, Google Scholar
40 : Entrainment of the circadian activity rhythm to the light cycle: effective light intensity for a Zeitgeber in the retinal degenerate C3H mouse and the normal C57BL mouse. Physiol Behav 1980; 24:523–527Crossref, Medline, Google Scholar
41 : Spectral sensitivity of a novel photoreceptive system mediating entrainment of mammalian circadian rhythms. Nature 1984; 308:186–188Crossref, Medline, Google Scholar
42 : Suppression of melatonin secretion in some blind patients by exposure to bright light. N Engl J Med 1995; 332:6–11Crossref, Medline, Google Scholar
43 : Circadian photoreception in the retinally degenerate mouse (rd/rd). J Comp Physiol A Neuroethol Sens Neural Behav Physiol 1991; 169:39–50Crossref, Google Scholar
44 : Spectral sensitivity of photoreceptors mediating phase-shifts of circadian rhythms in retinally degenerate CBA/J (rd/rd) and normal CBA/N (+/+)mice. J Comp Physiol A Neuroethol Sens Neural Behav Physiol 1996; 178:797–802Crossref, Google Scholar
45 : Regulation of mammalian circadian behavior by non-rod, non-cone, ocular photoreceptors. Science 1999; 284:502–504Crossref, Medline, Google Scholar
46 : Regulation of the mammalian pineal by non-rod, non-cone, ocular photoreceptors. Science 1999; 284:505–507Crossref, Medline, Google Scholar
47 : A novel human opsin in the inner retina. J Neurosci 2000; 20:600–605Crossref, Medline, Google Scholar
48 : Melanopsin and mechanisms of non-visual ocular photoreception. J Biol Chem 2012; 287:1649–1656Crossref, Medline, Google Scholar
49 : Human and macaque pupil responses driven by melanopsin-containing retinal ganglion cells. Vision Res 2007; 47:946–954Crossref, Medline, Google Scholar
50 : Melanopsin (Opn4) requirement for normal light-induced circadian phase shifting. Science 2002; 298:2213–2216Crossref, Medline, Google Scholar
51 : Role of melanopsin in circadian responses to light. Science 2002; 298:2211–2213Crossref, Medline, Google Scholar
52 : Action spectrum for melatonin regulation in humans: evidence for a novel circadian photoreceptor. J Neurosci 2001; 21:6405–6412Crossref, Medline, Google Scholar
53 : An action spectrum for melatonin suppression: evidence for a novel non-rod, non-cone photoreceptor system in humans. J Physiol 2001; 535:261–267Crossref, Medline, Google Scholar
54 : High sensitivity of the human circadian melatonin rhythm to resetting by short wavelength light. J Clin Endocrinol Metab 2003; 88:4502–4505Crossref, Medline, Google Scholar
55 : Short-wavelength light sensitivity of circadian, pupillary, and visual awareness in humans lacking an outer retina. Curr Biol 2007; 17:2122–2128Crossref, Medline, Google Scholar
56 : Short-wavelength sensitivity for the direct effects of light on alertness, vigilance, and the waking electroencephalogram in humans. Sleep 2006; 29:161–168Medline, Google Scholar
57 : Light therapy for seasonal affective disorder with blue narrow-band light-emitting diodes (LEDs). Biol Psychiatry 2006; 59:502–507Crossref, Medline, Google Scholar
58 : A controlled trial of the Litebook light-emitting diode (LED) light therapy device for treatment of seasonal affective disorder (SAD). BMC Psychiatry 2007; 7:38Crossref, Medline, Google Scholar
59 : The effects of blue-enriched light treatment compared to standard light treatment in seasonal affective disorder. J Affect Disord 2012; 136:72–80Crossref, Medline, Google Scholar
60 : Circadian phase delay induced by phototherapeutic devices. Aviat Space Environ Med 2007; 78:645–652Medline, Google Scholar
61 : A missense variant (P10L) of the melanopsin (OPN4) gene in seasonal affective disorder. J Affect Disord 2009; 114:279–285Crossref, Medline, Google Scholar
62 : The Power of Movement in Plants. London, Murray, 1880Crossref, Google Scholar
63 : Humoral phototransduction: blood is a messenger. Neuroscientist 1996; 2:207–210Crossref, Google Scholar
64 : New pathways in animal reproductive physiology frontiers and perspectives. J Physiol Pharmacol 1992; 43(suppl 1):5–19Medline, Google Scholar
65 : The gaseous messenger carbon monoxide is released from the eye into the ophthalmic venous blood depending on the intensity of sunlight. J Biol Regul Homeost Agents 2012; 26:111–118Medline, Google Scholar
66 : The oxygen tension of arterial blood. J Physiol 1896; 20:497–520Crossref, Medline, Google Scholar
67 : Photo-reversal by monochromatic light of the carbon monoxide-inhibited heme degradation catalyzed by the reconstituted heme oxygenase system. J Biochem 1981; 90:1671–1675Crossref, Medline, Google Scholar
68 : Melatonin is an efficient antioxidant. Arch Gerontol Geriatr 1995; 20:159–165Crossref, Medline, Google Scholar
69 : Über eine biologische Bedeutung der Gallenfarbstoffe: Bilirubin und Biliverdin als Antioxydantien für das Vitamin A und die essentiellen Fettsäuren. Helv Chim Acta 1954; 37:306–313Crossref, Google Scholar
70 : Ten-year-replicated circadian profiles for 36 physiological, serological and urinary variables in healthy men. Chronobiol Int 1988; 5:237–284Crossref, Medline, Google Scholar
71 : The diurnal variation of the serum iron concentration. Scand J Clin Lab Invest 1953; 5:118–121Crossref, Medline, Google Scholar
72 : The pineal gland: a model of neuroendocrine regulation, in The Hypothalamus. Edited by Reichlin SBaldessarini RJMartin JB. New York, Raven Press, 1978, pp 303–327Google Scholar
73 : Effects of light on low nocturnal bilirubin in winter depression: a preliminary report. Biol Psychiatry 2002; 51:422–425Crossref, Medline, Google Scholar
74 : Phototherapy for neonatal jaundice. N Engl J Med 2008; 358:920–928Crossref, Medline, Google Scholar
75 : Bilirubin and glutathione have complementary antioxidant and cytoprotective roles. Proc Natl Acad Sci USA 2009; 106:5171–5176Crossref, Medline, Google Scholar
76 : Quantum yields for laser photocyclization of bilirubin in the presence of human serum albumin: dependence of quantum yield on excitation wavelength. Photochem Photobiol 1989; 50:305–319Crossref, Medline, Google Scholar
77 : Formation of lumirubin during light therapy in adults. J Biol Sci 2004; 4:357–360Crossref, Google Scholar
78 : What a Plant Knows. New York, Scientific American, 2012Google Scholar
79 Adams F (ed): The Extant Works of Aretæus, the Cappadocian. London, Sydenham Society, 1856Google Scholar
80 : Retinal photodamage by endogenous and xenobiotic agents. Photochem Photobiol 2012; 88:1320–1345Crossref, Medline, Google Scholar