Thalamic and Prefrontal FDG Uptake in Never Medicated Patients With Schizophrenia
Abstract
OBJECTIVE: Because neuroleptic treatment may cause long-lasting changes in brain structure and function, a group of patients with schizophrenia who had never been medicated was recruited to examine regional glucose metabolic rates in the frontal-striato-thalamic circuit. METHOD: Twelve never medicated patients with schizophrenia (seven men, five women; mean age=29 years) and 13 normal volunteers (eight men and five women; mean age=28.5 years) underwent 18F-fluorodeoxyglucose (FDG) positron emission tomography, and coregistered anatomical magnetic resonance imaging scans were also obtained. During FDG uptake, subjects performed a spatial attention task previously shown to activate the pulvinar region of the thalamus. RESULTS: Diminished regional glucose metabolism was found in the medial dorsal nucleus, posterior thalamus, and prefrontal cortex of patients with schizophrenia relative to normal volunteers, extending earlier results from studies of medicated and previously medicated patients. CONCLUSIONS: The finding of lower relative metabolic rates in the frontothalamic circuits of patients with schizophrenia is consistent with extended circuit deficits involving interactions of frontal executive areas with thalamic sensory and association processes.
The prefrontal cortex, striatum, and thalamus form a neural circuit important in regulating sensory input, attention, and action. Deficits in these three areas in schizophrenia have been widely reported in both structural (1) and functional (2) brain imaging studies. The thalamus comprises multiple nuclei that relay and filter sensory and higher-order inputs to and from the cerebral cortex and limbic structures. Thus, it is a candidate structure for abnormality in schizophrenia, a disease that includes disturbed sensory and attentional function (3). Especially important within the thalamus may be the medial dorsal nucleus (with its interconnections with the prefrontal cortex) and the pulvinar (also interconnected with frontal and temporal regions). Both nuclei interact with the cortex in high-level cognitive activity and have been found to have reduced volumes in schizophrenia in postmortem as well as magnetic resonance imaging (MRI) studies (4–6).
In our earliest positron emission tomography (PET) studies, which used 18F-fluorodeoxyglucose (FDG) and employed older-generation PET scanners with lesser in-plane resolutions of 15 mm (7) or 7.5 mm (8), we did not find decreased metabolic rates in the thalamus of unmedicated (previously medicated) schizophrenia patients compared with healthy subjects. In a study of never medicated schizophrenia subjects, we found less prominent regional glucose metabolic rate (rGMR) in the mediodorsal nucleus region (6). In another study of never medicated patients (9), blood flow decreases in the thalamus, frontal lobe, and temporal lobe were identified. With increased spatial PET resolution and coregistered structural MRI (1.2-mm thick slices) scans obtained for the entire thalamic volume, lower rGMR in the medial dorsal nucleus of unmedicated schizophrenia patients relative to healthy subjects was statistically confirmed, although whole thalamus metabolism did not differ between groups (10). However, when the nuclei were traced on coregistered MRI templates, decreased metabolic rates in the medial dorsal and centromedian nuclei (11), but not the pulvinar, were confirmed. Decreased rGMR in the remainder of the thalamus was again not found, indicating specificity of the effect to the medial association regions. That study, while large (61 normal subjects and 40 patients), was limited in having patients who, although unmedicated, had previously been treated with neuroleptics, which might have affected thalamic volume and rGMR. In addition, the uptake condition was the serial verbal learning task, which activates the frontal lobe but is not known to activate the pulvinar.
In the current study we selected a specific task known to activate the pulvinar (12) for the uptake condition and recruited patients who had never been medicated. We have undertaken two separate, independent coregistration methods to replicate thalamic effects and used internal brain fiducial landmarks to assess coregistration accuracy and alignment in the 12-point affine transformation to standard space.
Method
Subjects
A group of 12 psychotic patients (seven men and five women; mean age=29.0 years [SD=9.8, range=20–46]) was recruited from the greater Dayton, Ohio, area and was evenly divided between inpatients and outpatients. After complete description of the study, all subjects completed a verbal “informed consent post test.” All participants passed this test and gave written informed consent. Subjects were evaluated with the Comprehensive Assessment of Symptoms and History (13), 18-item version of the Brief Psychiatric Rating Scale (BPRS) (14), and the Abnormal Involuntary Movement Scale (AIMS) (15) and were given their diagnosis by a psychiatrist (D.S.L.) who used DSM-IV criteria. Patients had either never been medicated (N=11) or almost never medicated (one subject had a lifetime neuroleptic exposure of no more than five doses several years before this study evaluation). Subject characteristics are summarized in Table 1. Following study evaluation, all subjects were immediately referred for psychiatric treatment.
Thirteen healthy comparison subjects (eight men and five women; mean age=28.5 years [SD=8.0, range=19–41]) were age- and sex-matched to the experimental subjects. Informed consent was obtained as described earlier. Comparison subjects were assessed by a psychiatrist (D.S.L.). Participating subjects had no history of psychiatric illness, substance use disorder, clinically significant head trauma, or active neurological disease. Comparison subjects did not significantly differ from ill subjects with respect to race (all but three healthy subjects and one schizophrenia patient were white), handedness (all but one healthy subject were right-handed), years of education (mean=14.5 [SD=2.2] and 13.7 [SD=2.4], respectively), or family-of-origin socioeconomic status (mean Hollingshead Two-Factor Index of Social Position [16] score=34.6 [SD=7.0] and 31.3 [SD=19.9]).
All subjects fasted for at least 4 hours before reporting to the PET department. Blood glucose determination was performed before FDG administration, and all subjects had a normal blood glucose level (healthy subjects: mean=83.9 mg/dl [SD=8.8, range=66–98]; schizophrenia patients: mean=86.5 mg/dl [SD=8.2, range=74–100]).
PET Scans
FDG was administered intravenously (mean=7.8 mCi, SD=0.6). Emission scanning commenced 40 minutes following the injection of FDG. The data were acquired with an ECAT EXACT HR+ PET scanner in three-dimensional mode (17). Subjects were positioned in the PET camera with the canthomeatal line parallel to the in-plane field of view. Before acquisition of the FDG data, a 68Ge/68Ga transmission scan was acquired for 5 minutes to correct for the attenuation of radiation. A single emission scan of 20 minutes’ duration was acquired. The data were reconstructed by using ECAT version 7.2 software implementation of filtered backprojection (4-mm Hann filter) with a pixel size of 1.84×1.84×2.4 mm. The average interval between FDG injection and commencement of PET scanning was 45.04 minutes (SD=1.51), with no difference between healthy subjects (mean=44.64 minutes [SD=1.39, range=40–46]) and schizophrenia patients (mean=45.55 minutes [SD=1.57, range=44–50]).
MRI Scans
T1-weighted axial MRI scans were acquired with the GE Signa 5x system (General Electric, Milwaukee) (TR=24 msec, TE=5 msec, flip angle=40°, slice thickness=1.2 mm, pixel matrix=256×256, field of view=23 cm, total slices=128). The interval between FDG PET and MRI scans averaged approximately 1 day for schizophrenia patients (mean=0.92 days, range=0–3) and 2 weeks for comparison subjects (mean=13.6 days, range=3–70).
Uptake Condition
During the 40-minute FDG uptake period, all subjects carried out a visual attention task that required separation of target stimuli from competing surroundings as in our earlier PET study (12). Subjects visually fixated upon a dot corresponding to the center of the screen then looked at stimuli positioned horizontally at 2° to the right or to the left of the central fixation point. The target stimulus (the letter O) either appeared alone as an upper-case character or as a lower-case character surrounded by eight other letters (Figure 1). In half of the trials, the letter C or the digit zero (0) were presented as distracters. The subject’s task was to click on the mouse each time he detected the letter O either alone or surrounded by small letters, ignoring the C and the 0, and to press on the right button for a right-sided target and the left button for a left-sided target. The overall size of the stimuli was controlled so that the big letters were of the same dimensions as the pattern of small letters surrounded by flankers (i.e., each stimulus display was 19×22 mm). Each display was flashed for 150 msec. There were four experimental runs, each 264 seconds in duration, with a brief rest interval of 24 seconds between each session. Each run began with a 24-second period of blank screen followed by a block of 12 display stimuli, eight of one type and four drawn at random from the other three types to maintain expectancy. The four display types were large letter in 1) left or 2) right hemifield and small letter with flankers in 3) left or 4) right hemifield. The order of runs was counterbalanced across subjects. The subjects were signaled with a blue flash in case of a miss or an error. Subjects were trained before FDG administration.
Data Analysis
Significance probability maps of the datasets were created using two complementary methods, statistical parametric mapping software (SPM) (18) and the FMRIB Software Library (FSL), version 5.00 (www.fmrib.ox.ac.uk/fsl). We chose SPM2 to survey the entire smoothed brain and FSL with our own R programs to evaluate the primary brain region, the thalamus with unsmoothed MRI template coregistered images. For SPM2, the FDG images were first spatially normalized to the FDG template defined with the SPM 99 software (and subsequently converted for SPM2) using the default parameters for spatial normalization, adjustment for whole brain metabolic rate by covariance, and 8-mm smoothing. Both SPM and FSL data are unitless. The normalized images were then closely inspected to ensure proper alignment. A schizophrenia and healthy group comparison was made by using the two-sample t test criterion. The t test results were then displayed on the FDG template. For closer examination of the thalamus, we used FSL processing. MRI anatomical images had the brain extracted from the skull with the Brain Extraction Tool (19) and were placed in standard position (anterior and posterior commissures in the same plane, midline x=0, vertical level z=0) using a 6-parameter transform. No images were smoothed by filtering using the FSL method. The FDG images were coregistered to each individual’s anatomical image again with the 6-parameter transform and the FMRIB Linear Image Registration Tool (20). Next, each person’s MRI anatomical images were transformed to Montreal Neurological Institute (MNI) coordinates with a 12-point transformation and the transformation matrix used to similarly transform the unsmoothed FDG images, which were then divided by the whole brain average metabolic rate. The FDG images then had pixel-by-pixel t tests computed using our own program in the R computer language (http://www.r-project.org). Since we had already reported medial dorsal nucleus FDG decreases in two earlier and entirely independent samples (11), both predicting the finding in advance of SPM application, images were thresholded at p<0.05. This provides an alternate coregistration method unbiased by systematic group differences in brain rGMR, since the FDG itself did not enter the coregistration transformations. The FDG data was unsmoothed (in the FSL analysis) for maximum spatial resolution in a priori hypothesis testing. While SPM maps are typically corrected for multiple comparisons because of their exploratory nature, this analysis was confirmatory of our earlier FDG studies and neuroanatomical studies of the medial dorsal nucleus.
We examined the quality of the FMRIB Linear Image Registration Tool coregistration and standardization to MNI space on the final MNI FDG and anatomical images. We chose the horizontal slice at z=12 because it was close to the center of the medial dorsal nucleus. Three regions were chosen as internal fiducial marks: 1) along the x=0 midline, the transition from the gray matter of the posterior cingulate (Brodmann’s areas 29, 30) to the white matter of the corpus callosum; 2) along the x=–4 anteroposterior line, the transition from the gray matter of areas 24 and 32 to the white matter or the genu of the corpus callosum; and 3) the fluid space between the two halves of the posterior thalamus along the y=–24 line. These landmarks were chosen because 1) there would be a rapid change in values along a unidimensional column of pixels for both PET (from high values in gray to low values in white) and for MRI (from low values in gray to high values in white) and 2) they were adjacent to the medial dorsal nucleus. The medial dorsal nucleus itself is more difficult to trace, and intertracer variability would also have been involved in its use as an internal landmark. Both PET and MRI would be expected to have low values in the midline position chosen. The PET and MRI values were differentiated (xn–xn+1) and the location of the maximum value determined. This location should be the same for every MNI standardized brain and appear at the same location in every MNI standardized PET. Variation in the quality of the 12-point affine standardization and its success in aligning all structures can be assessed by examining the MNI MRI data. The MNI images were examined in MRIcro, and an R program created in house by one of the authors (M.S.B.) was used to calculate the first derivative strips. The MNI pixel size was 2 mm. The posterior cingulate landmark had a mean y=–46.5, and 85% of subjects fell from –45 to –49, an error of one discrete pixel. The Talairach atlas shows this location at y=–44. The midline was found on the MRI at x=0 in 62% and an error of one pixel on either side (–2 or 2) in 92%. The midline was found on PET at x=0 in 38% and within an error of one pixel on either side in 100%. The differences in distance between the location of maximum first derivative points on MRI and PET were 0 pixels for 57% of subjects and within one pixel error for 71%. The anterior cingulate landmark was somewhat more variable anatomically with a mean of 26.7, 71% of subjects within the one pixel error, and the Talairach atlas position y=30. The differences between the location of maximum first derivative points between MRI and FDG were 0 for 53% and within one pixel for 85%. Thus, the combined error of brain standardization and coregistration (PET locations) was within one pixel (2 mm) in 80%–90% of landmark examinations and adequate for regional examination of the thalamus. This compares favorably with the typical width of the medial dorsal nucleus, 6–8 mm. For comparison, the thalamus is 21 mm wide (x) and 30 mm long (y) at this level; a medial dorsal nucleus bounding box in the Talairach atlas at this level (z=12) would be 9 mm in the x dimension and 13 mm in the y dimension.
The thalamus and medial dorsal nucleus were also assessed using a stereotaxic region of interest atlas approach for confirmation (21). This method has stored coordinates for MNI brain structures including the thalamus and the medial dorsal nucleus.
Last, one of the authors (E.M.K.) traced the whole thalamus, medial dorsal nucleus, and pulvinar on raw unresliced MRI templates exactly as previously reported (4, 5). FDG images were coregistered to these anatomical images as before. Relative metabolic rates within these areas and the whole thalamus, not including the medial dorsal nucleus, were obtained using a Matlab routine.
Results
SPM Mapping
Patients with schizophrenia had lower relative metabolic rates in the thalamus and lateral prefrontal cortex, especially at the orbitofrontal levels (Figure 2). A significant reduction in metabolic rate was also noted in additional cortical areas, including the insula, temporal lobes, parietal lobe (Brodmann’s area 39), and the anterior cingulate at its most dorsal level (Brodmann’s area 24) (22). The posterior putamen was also less active in the schizophrenia subjects. Small regions in the temporal poles and medial temporal lobe are less prominently represented than the prefrontal cortex among areas significantly lower in patients with schizophrenia. No gray matter areas were found significantly higher in patients with schizophrenia than healthy comparison subjects.
Pixel-by-Pixel Examination of the Thalamus
Coregistration is illustrated in Figure 3, and detailed examination of Talairach z=12 slice in Figure 4. The red area in Figure 4 indicates pixels where the healthy subjects have higher relative metabolic rates than patients with schizophrenia (p<0.05, two-tailed), which extends across the region of the medial dorsal nucleus and pulvinar.
Stereotaxic Region-of-Interest Examination of the Thalamus
The medial dorsal nucleus had a relative metabolic rate significantly higher in healthy volunteers (mean=11.8, SD=0.64) than patients with schizophrenia (mean=11.2, SD=0.64) (t=2.88, df=23, p=0.008). The whole thalamus did not differ between normal subjects and patients (mean=10.0 [SD=0.43] and 9.7 [SD=0.49], respectively). Repeated measures two-way analysis of variance (ANOVA) with whole thalamus and medial dorsal nucleus for the right and left hemisphere revealed a significant diagnostic group difference (F=6.66, df=1, 23, p<0.02) and a significant interaction between group and region of interest (whole thalamus, medial dorsal nucleus alone)(F=6.45, df=1, 23, p<0.02), indicating that the metabolic group difference effect for the medial dorsal nucleus was significantly greater than for the whole thalamus. There was no significant diagnostic group-by-hemisphere or diagnostic group-by-hemisphere-by-structure interaction.
MRI Template-Traced Nuclei
A lower metabolic rate in the medial dorsal nucleus for patients with schizophrenia (mean=2.59, SD=0.29) versus healthy subjects (mean=2.76, SD=0.28) was confirmed (F=4.62, df=1, 18, p<0.05), and this was specific to the medial dorsal region when compared with the remainder of the thalamus in a three-way ANOVA (diagnostic group [healthy, schizophrenia] by structure [medial dorsal, remainder of thalamus] by hemisphere [right, left]), with a significant group-by-structure interaction (F=5.51, df=1, 18, p<0.04). It is of interest that all subjects except one schizophrenia patient had higher relative values in the medial dorsal nucleus than in the remainder of the thalamus. While the relative metabolic rate was lower in the pulvinar in patients with schizophrenia, this difference did not reach statistical significance. The correlation between the stereotaxic medial dorsal nucleus and the traced medial dorsal nucleus was 0.51.
Task Performance
All healthy subjects and all but one patient performed the task above chance levels and completed responses in every trial block during the uptake period. Removal of the one schizophrenia subject who did not perform the task did not alter the significance of the group comparison t test on the medial dorsal nucleus (t=2.31, df=22, p=0.03) or the diagnostic group difference with ANOVA (F=4.32, df=1, 22, p<0.05).
Discussion
Our results confirm earlier studies that reported relative metabolic rate reduction in the thalamus and lateral prefrontal cortex in patients with schizophrenia, extending those findings in several important ways. We have demonstrated these findings in a group of never medicated patients, indicating that these reductions could not be due to medication artifacts. Further, the use of an uptake condition demonstrated to have thalamic activation in earlier PET studies enhances the strength of the conclusions. Replicating the results with methodologically complementary pixel-by-pixel t test methods and traced templates allows both whole-brain exploration and the specific regions to be exhaustively tested. These specific methodological steps appear to have enhanced the power of the FDG PET images to confirm group differences in comparison with some earlier studies that used medicated patients, an eyes-closed resting condition, or lower-resolution image acquisition.
The limitations of our present study include the relatively modest number of never medicated subjects, image resolution and coregistration quality, and the use of relative metabolic rates. While somewhat smaller than two earlier studies of never medicated patients (6, 9), we had adequate power to detect differences in thalamic rGMR. Methods are currently being explored to overcome image resolution limitations by applying a partial volume correction to the image data. However, this correction has not been fully validated and has the potential to introduce a large bias into the analysis (23, 24). With a structure as small as the thalamus or medial dorsal nucleus (6–9 mm wide), image coregistration and the quality of structure position standardization are critical. We evaluated joint error in our examination of FDG image coordinates and found that about 75% of subjects’ FDG landmarks adjacent to the medial dorsal nucleus lay within one 2-mm pixel of a standard location and of the matching MRI template. Last, we thresholded the significance probability images at the p<0.05 level without correction. This lacks correction for multiple t testing, but since the study was focused specifically on the medial dorsal region (the relative metabolic rate of which was previously reported to be reduced [11]), and the dorsolateral prefrontal cortex was previously explored with significance probability mapping (25), it seemed appropriate to consider the maps as confirmatory rather than exploratory. The medial dorsal region selected a priori and assessed using stereotaxic position also yielded a p=0.0083 probability (0.0041 one-tailed in replication). While absolute metabolic rates for the medial dorsal nucleus could have been obtained with the FDG method, there is no evidence that total brain metabolic rate differs between normal subjects and patients with schizophrenia. Thus the small regions of thalamic and prefrontal metabolism reductions seen in Figure 2 seem unlikely to have artifactually arisen on the basis of other large areas of cortical metabolic rate shifts.
One might argue that patients with schizophrenia lacked motivation to perform the task and that differences in the medial dorsal nucleus were an indirect artifact of diminished involvement in successful task performance, rather than an indication of primary regional pathology. Against this is the fact that all but one patient participated in the task and performed well above chance levels (results were unchanged after omission of the one nonperforming patient). Further, motivational areas (Brodmann’s areas 11, 12) and vigilance areas (Brodmann’s area 32) did not show significant decrease or increase, suggesting that failure in nonspecific task engagement regions is not entirely responsible for the medial dorsal nucleus deficit. It is interesting that patients with obsessive-compulsive disorder may have higher blood flow in their thalamus (26). Thus, potential reciprocal interactions between spatial attention (medial dorsal nucleus) and motivation (orbital and medial prefrontal cortex), the impairment of which is a central feature of the schizophrenia syndrome, cannot be ruled out.
Our use of unmedicated and previously untreated patients is important in relating the findings to schizophrenia rather than medication effects. Studies have reported decreases in relative metabolic rate in the frontal lobe with clozapine (27) and haloperidol (28) but not with risperidone (29), so the use of unmedicated patients is especially important for establishing this finding. It is interesting that patients with higher dorsolateral prefrontal metabolic rates were reported likely to respond to clozapine (30).
Other studies have also confirmed reduced thalamic FDG uptake in schizophrenia (31, 32) (reviewed elsewhere [11]). Last, using functional MRI (fMRI), reduced activation in the thalamus has been observed. The anterior thalamic region as well as the medial and anterior frontal lobe had reduced fMRI blood oxygenation level-dependent activation in medicated patients with schizophrenia during the continuous performance test of vigilance (33), both areas adjacent to but not exactly overlapping with our regions. Less thalamic activation in schizophrenia was also seen in other fMRI studies (34). However, significant thalamic activation was observed in medicated patients with schizophrenia but not in normal subjects during performance of the Sternberg memory task (35), although apparently no direct map of group differences in activation was presented.
Examination of the pixel-by-pixel maps indicates that medial and posterior portions of the thalamus appear to be the main regions of the thalamus with decreased metabolic rates. The thalamus comprises multiple nuclei that relay and filter sensory and higher-order inputs to and from the cerebral cortex and limbic structures (3). The biggest thalamic substructures, the medial dorsal nucleus and the pulvinar (both visible on MRI), are of particular interest in attention because of their reciprocal connections with the prefrontal and temporal regions and because their size and metabolic rate appear diminished in imaging studies of patients with schizophrenia. The medial dorsal nucleus has strong interconnections with the dorsolateral prefrontal cortex (36), and the connections of the medial dorsal nucleus have been used to define the prefrontal cortex (37), a key area of executive action and focusing of attention thought to be defective in schizophrenia (reviewed by Buchsbaum and Hazlett [2]). Crosson (38) suggests the medial dorsal nucleus as a critical element in an attentional “selective engagement” system that impacts semantic functions in schizophrenia.
It should be noted that the use of never medicated patients may be especially important in establishing regional metabolic differences in the thalamus and that even thalamic volume may be affected by neuroleptics (39). The recent report of decreased thalamic D2 receptor binding in the medial portion of the thalamus in drug-naive patients with schizophrenia (40) indicates that both chronic and acute medication effects could influence thalamic metabolic rate. That important study not only supports a role for the medial thalamus in schizophrenia but also suggests that the medial regions of the thalamus are potentially informative for understanding medication effects as well.
Until the mid-1970s it was thought that the prefrontal cortex received its thalamic input solely from the medial dorsal nucleus; however, it is now apparent that the pulvinar also contributes to its innervation (41, 42). The pulvinar, important in visual and possibly auditory attention (43–45), also has prominent interconnections with the parietal and temporal lobe. The current findings of decreased metabolic rate in the medial and posterior regions of the thalamus, prefrontal cortex, and posterior temporal and parietal regions tend to confirm a network of frontothalamic and parieto-temporal-thalamic rGMR concurrently diminished in patients carrying out a task demonstrated to activate the pulvinar (12).
Much work in the field of visual neurophysiology suggests that the pulvinar signals the importance or relevance of stimuli that fall inside classically defined visual receptive fields (43). Neurophysiological studies in animals have found that neurons of the pulvinar respond specifically to stimuli to be the target of a saccade (46). These findings suggest that the pulvinar may play a role in “visual salience,” that is, the attention-related selection of a target (44), and “directed attention” (12). The anatomy and projections of the pulvinar suggest functional cortical-thalamic-cortical loops involved in a variety of functions including salience, attention, and working memory (43). The fact that both the medial dorsal nucleus and the pulvinar express D2 type dopamine receptors (40), together with their demonstrated role in selective attention, fits well with recent formulations of schizophrenia as a hyperdopaminergic state that leads to an aberrant assignment of salience to elements of one’s experience (47). According to that model, both typical and atypical antipsychotics “dampen the salience” of abnormal experiences via blockade of D2 receptors and by doing so, allow the resolution of symptoms.
![]() |
Received March 25, 2004; revision received June 7, 2004; accepted June 14, 2004. From the Wallace-Kettering Neuroscience Institute. Address correspondence and reprint requests to Dr. Lehrer, Wright State University Department of Psychiatry, c/o The Wallace-Kettering Neuroscience Institute, 3533 Southern Blvd., Suite 5200, Kettering, OH 45429; [email protected] (e-mail). Major support was provided by Wallace-Kettering Neuroscience Institute. Use of imaging resources supported by the United States Air Force, Air Force Research Laboratory (AFRL/HEOP), Air Force Materiel Command, under cooperative agreement F33615-98-2-6002. The authors thank Tonya Perkins, Marilyn Brackney, Kelly Dunigan, Kerry Kovacs, Yuliya Lelchuk, and Drs. Jogesh Mukherjee, Martin Satter, and Kingwai Chu for technical assistance. The authors also thank Drs. Bing Shi, T.K. Narayanan, and Steve Mattmuller for radiopharmaceutical production.
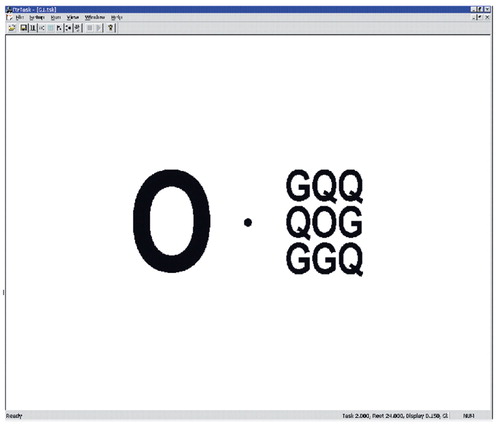
Figure 1. Stimulus Display Elements of the Visual Attention Task Given to Subjects During FDG Uptake
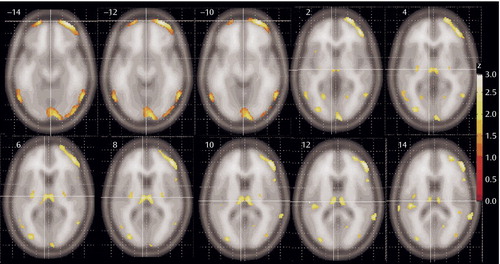
Figure 2. Regions of Significantly Different Relative Metabolic Rate Between Never Medicated Patients With Schizophrenia and Healthy Comparison Subjectsa
aColor bar shows z level. Note regions in thalamus (pulvinar and medial dorsal regions), frontal cortex (Brodmann’s areas 11, 10, 46, and 47), and temporal cortex (Brodmann’s areas 20, 21, and, to a limited extent, area 22). The posterior putamen was also less active in ill subjects. The centers of the thalamic regions were at –6, –16, 8 and at 6, –16, 8 (total volume=297 voxels, zmax=2.37 and 2.32, respectively).
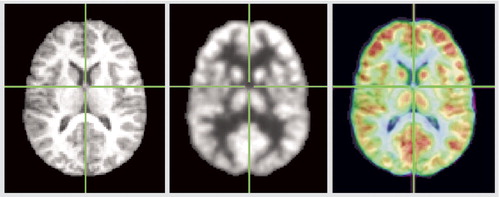
Figure 3. MNI Spatial Normalization and Coregistration With Fusion Image
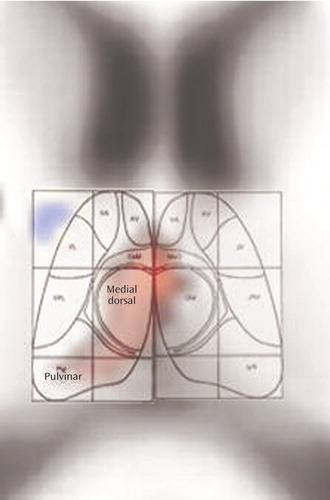
Figure 4. Detailed Examination of Thalamic Slice Depicting Metabolic Rate Differences Between Never Medicated Patients With Schizophrenia and Healthy Comparison Subjectsa
aArea in red indicates pixels in which two-tailed t tests confirmed significantly higher values (p<0.05) in healthy subjects relative to patients with schizophrenia. Background image of MNI brain has been secondarily enlarged with trilinear interpolation after all statistical computations were made (Photoshop). Region of thalamus overlaid with drawing of thalamic nuclei developed by D. LaBerge.
1. Shenton ME, Dickey CC, Frumin M, McCarley RW: A review of MRI findings in schizophrenia. Schizophr Res 2001; 49:1–52Crossref, Medline, Google Scholar
2. Buchsbaum M, Hazlett E: Positron emission tomography studies of abnormal glucose metabolism in schizophrenia. Schizophr Bull 1998; 24:343–364Crossref, Medline, Google Scholar
3. Jones E: Cortical development and thalamic pathology in schizophrenia. Schizophr Bull 1997; 23:483–501Crossref, Medline, Google Scholar
4. Byne W, Buchsbaum MS, Kemether E, Hazlett EA, Shinwari A, Mitropoulou V, Siever LJ: Magnetic resonance imaging of the thalamic mediodorsal nucleus and pulvinar in schizophrenia and schizotypal personality disorder. Arch Gen Psychiatry 2001; 58:133–140Crossref, Medline, Google Scholar
5. Kemether EM, Buchsbaum MS, Byne W, Hazlett EA, Haznedar M, Brickman AM, Platholi J, Bloom R: Magnetic resonance imaging of mediodorsal, pulvinar, and centromedian nuclei of the thalamus in patients with schizophrenia. Arch Gen Psychiatry 2003; 60:983–991Crossref, Medline, Google Scholar
6. Buchsbaum MS, Someya T, Teng CY, Abel L, Chin S, Najafi A, Haier RJ, Wu J, Bunney WE Jr: PET and MRI of the thalamus in never-medicated patients with schizophrenia. Am J Psychiatry 1996; 153:191–199Link, Google Scholar
7. Buchsbaum M, Ingvar D, Kessler R, Waters R, Cappelletti J, van Kammen D, King A, Johnson J, Manning R, Flynn R, Mann L, Bunney WJ, Sokoloff L: Cerebral glucography with positron tomography. Arch Gen Psychiatry 1982; 39:251–259Crossref, Medline, Google Scholar
8. Siegel BV Jr, Buchsbaum MS, Bunney WE Jr, Gottschalk LA, Haier RJ, Lohr JB, Lottenberg S, Najafi A, Nuechterlein KH, Potkin SG, Wu J: Cortical-striatal-thalamic circuits and brain glucose metabolic activity in 79 unmedicated male schizophrenics. Am J Psychiatry 1993; 150:1325–1336Link, Google Scholar
9. Andreasen NC, O’Leary DS, Flaum M, Nopoulos P, Watkins GL, Boles Ponto LL, Hichwa RD: Hypofrontality in schizophrenia: distributed dysfunctional circuits in neuroleptic-naive patients. Lancet 1997; 349:1730–1734Crossref, Medline, Google Scholar
10. Hazlett EA, Buchsbaum MS, Byne W, Wei T-C, Spiegel-Cohen J, Geneve C, Kinderlehrer R, Haznedar MM, Shihabuddin L, Siever LJ: Three-dimensional analysis with MRI and PET of the size, shape, and function of the thalamus in the schizophrenia spectrum. Am J Psychiatry 1999; 156:1190–1199Abstract, Google Scholar
11. Hazlett EA, Buchsbaum MS, Kemether E, Bloom R, Platholi J, Brickman AM, Shihabuddin L, Tang C, Byne W: Abnormal glucose metabolism in the mediodorsal nucleus of the thalamus in schizophrenia. Am J Psychiatry 2004; 161:305–314Link, Google Scholar
12. LaBerge D, Buchsbaum MS: Positron emission tomographic measurements of pulvinar activity during an attention task. J Neurosci 1990; 10:613–619Crossref, Medline, Google Scholar
13. Andreasen NC, Flaum M, Arndt S: The Comprehensive Assessment of Symptoms and History (CASH): an instrument for assessing diagnosis and psychopathology. Arch Gen Psychiatry 1992; 49:615–623Crossref, Medline, Google Scholar
14. Overall JE, Gorham DR: The Brief Psychiatric Rating Scale. Psychol Rep 1962; 10:799–812Crossref, Google Scholar
15. Kane JM, Schooler NR: Tardive Dyskinesia Workgroup report. Psychopharmacol Bull 1980; 16:35–36Medline, Google Scholar
16. Hollingshead AB, Redlich FC: Social Class and Mental Illness: A Community Study. New York, John Wiley & Sons, 1958Google Scholar
17. Brix G, Zaers J, Adam LE, Bellemann ME, Ostertag H, Trojan H, Haberkorn U, Doll J, Oberdorfer F, Lorenz WJ: Performance evaluation of a whole-body PET scanner using the NEMA protocol. J Nucl Med 1997; 38:1614–1623Medline, Google Scholar
18. Friston K, Holmes A, Worsley K, Poline J, Frith C, Frackowiak R: Statistical parametric maps in functional imaging: a general linear approach. Hum Brain Mapp 1995; 2:189–210Crossref, Google Scholar
19. Smith SM: Fast robust automated brain extraction. Hum Brain Mapp 2002; 17:143–155Crossref, Medline, Google Scholar
20. Jenkinson M, Smith S: A global optimisation method for robust affine registration of brain images. Med Image Anal 2001; 5:143–156Crossref, Medline, Google Scholar
21. Maldjian JA, Laurienti PJ, Kraft RA, Burdette JH: An automated method for neuroanatomic and cytoarchitectonic atlas-based interrogation of fMRI data sets. Neuroimage 2003; 19:1233–1239Crossref, Medline, Google Scholar
22. Devinsky O, Morrell M, Vogt B: Contributions of anterior cingulate cortex to behavior. Brain 1995; 118:279–306Crossref, Medline, Google Scholar
23. Sossi V: Correction for image degrading factors is essential for accurate quantification of brain function using PET: against the proposition. Med Phys 2004; 31:425–426Google Scholar
24. Zaidi H. Correction for image degrading factors is essential for accurate quantification of brain function using PET: for the proposition. Med Phys 2004; 31:423–425Crossref, Medline, Google Scholar
25. Buchsbaum MS, Hazlett EA, Haznedar MM, Spiegel-Cohen J, Wei TC: Visualizing fronto-striatal circuitry and neuroleptic effects in schizophrenia. Acta Psychiatr Scand Suppl 1999; 395:129–137Crossref, Medline, Google Scholar
26. Lacerda AL, Dalgalarrondo P, Caetano D, Camargo EE, Etchebehere EC, Soares JC: Elevated thalamic and prefrontal regional cerebral blood flow in obsessive-compulsive disorder: a SPECT study. Psychiatry Res 2003; 123:125–134Crossref, Medline, Google Scholar
27. Potkin SG, Buchsbaum MS, Jin Y, Tang C, Telford J, Friedman G, Lottenberg S, Najafi A, Gulasekaram B, Costa J, et al.: Clozapine effects on glucose metabolic rate in striatum and frontal cortex. J Clin Psychiatry 1994; 55(suppl B):63–66Google Scholar
28. Desco M, Gispert JD, Reig S, Sanz J, Pascau J, Sarramea F, Benito C, Santos A, Palomo T, Molina V: Cerebral metabolic patterns in chronic and recent-onset schizophrenia. Psychiatry Res 2003; 122:125–135Crossref, Medline, Google Scholar
29. Molina V, Gispert JD, Reig S, Sanz J, Pascau J, Santos A, Palomo T, Desco M: Cerebral metabolism and risperidone treatment in schizophrenia. Schizophr Res 2003; 60:1–7Crossref, Medline, Google Scholar
30. Molina V, Reig S, Pascau J, Sanz J, Sarramea F, Gispert JD, Luque R, Benito C, Palomo T, Desco M: Anatomical and functional cerebral variables associated with basal symptoms but not risperidone response in minimally treated schizophrenia. Psychiatry Res 2003; 124:163–175Crossref, Medline, Google Scholar
31. Paradiso S, Andreasen NC, Crespo-Facorro B, O’Leary DS, Watkins GL, Boles Ponto LL, Hichwa RD: Emotions in unmedicated patients with schizophrenia during evaluation with positron emission tomography. Am J Psychiatry 2003; 160:1775–1783Link, Google Scholar
32. Clark C, Kopala L, Li DK, Hurwitz T: Regional cerebral glucose metabolism in never-medicated patients with schizophrenia. Can J Psychiatry 2001; 46:340–345Crossref, Medline, Google Scholar
33. Volz H, Gaser C, Hager F, Rzanny R, Ponisch J, Mentzel H, Kaiser WA, Sauer H: Decreased frontal activation in schizophrenics during stimulation with the Continuous Performance Test—a functional magnetic resonance imaging study. Eur Psychiatry 1999; 14:17–24Crossref, Medline, Google Scholar
34. Kumari V, Gray JA, Geyer MA, ffytche D, Soni W, Mitterschiffthaler MT, Vythelingum GN, Simmons A, Williams SC, Sharma T: Neural correlates of tactile prepulse inhibition: a functional MRI study in normal and schizophrenic subjects. Psychiatry Res 2003; 122:99–113Crossref, Medline, Google Scholar
35. Manoach DS, Gollub RL, Benson ES, Searl MM, Goff DC, Halpern E, Saper CB, Rauch SL: Schizophrenic subjects show aberrant fMRI activation of dorsolateral prefrontal cortex and basal ganglia during working memory performance. Biol Psychiatry 2000; 48:99–109Crossref, Medline, Google Scholar
36. Giguere M, Goldman-Rakic PS: Mediodorsal nucleus: areal, laminar, and tangential distribution of afferents and efferents in the frontal lobe of rhesus monkeys. J Comp Neurol 1988; 277:195–213Crossref, Medline, Google Scholar
37. Rose J, Woolsey C: The orbitofrontal cortex and its connections with the mediodorsal nucleus in rabbit, sheep, and cat. Assoc Res Nerv Ment Dis Proc 1948; 27:210–282Medline, Google Scholar
38. Crosson B: Subcortical mechanisms in language: lexical-semantic mechanisms and the thalamus. Brain Cogn 1999; 40:414–438Crossref, Medline, Google Scholar
39. Strungas S, Christensen JD, Holcomb JM, Garver DL: State-related thalamic changes during antipsychotic treatment in schizophrenia: preliminary observations. Psychiatry Res 2003; 124:121–124Crossref, Medline, Google Scholar
40. Talvik M, Nordstrom AL, Olsson H, Halldin C, Farde L: Decreased thalamic D2/D3 receptor binding in drug-naive patients with schizophrenia: a PET study with [11C]FLB 457. Int J Neuropsychopharmacol 2003; 6:361–370Crossref, Medline, Google Scholar
41. Goldman-Rakic PS, Porrino LJ: The primate mediodorsal (MD) nucleus and its projection to the frontal lobe. J Comp Neurol 1985; 242:535–560Crossref, Medline, Google Scholar
42. Romanski LM, Giguere M, Bates JF, Goldman-Rakic PS: Topographic organization of medial pulvinar connections with the prefrontal cortex in the rhesus monkey. J Comp Neurol 1997; 379:313–332Crossref, Medline, Google Scholar
43. Grieve KL, Acuna C, Cudeiro J: The primate pulvinar nuclei: vision and action. Trends Neurosci 2000; 23:35–39Crossref, Medline, Google Scholar
44. Robinson DL: Functional contributions of the primate pulvinar. Prog Brain Res 1993; 95:371–380Crossref, Medline, Google Scholar
45. Wester K, Irvine DR, Hugdahl K: Auditory laterality and attentional deficits after thalamic haemorrhage. J Neurol 2001; 248:676–683Crossref, Medline, Google Scholar
46. Benevento LA, Port JD: Single neurons with both form/color differential responses and saccade-related responses in the nonretinotopic pulvinar of the behaving macaque monkey. Vis Neurosci 1995; 12:523–544Crossref, Medline, Google Scholar
47. Kapur S: Psychosis as a state of aberrant salience: a framework linking biology, phenomenology, and pharmacology in schizophrenia. Am J Psychiatry 2003; 160:13–23Link, Google Scholar