Effects of Ketamine on Leading Saccades During Smooth-Pursuit Eye Movements May Implicate Cerebellar Dysfunction in Schizophrenia
Abstract
OBJECTIVE: Ketamine has proved a useful probe in the study of schizophrenia. Recent studies have shown that ketamine causes abnormalities in eye tracking similar to those seen in patients with schizophrenia. The authors examined the effects of ketamine on leading saccadic eye movements, a specific component of the smooth-pursuit response shown to be abnormal in schizophrenia patients and their relatives. METHOD: Twelve normal healthy volunteers received a 0.1 mg/kg bolus injection of ketamine or placebo in double-blind fashion during a smooth-pursuit eye-movement task. The number of leading saccades and the ratios of leading saccades to smooth-pursuit response time and to total saccadic eye-movement time were measured. RESULTS: Ketamine significantly increased the number of leading saccades and increased the leading saccade ratios for more slowly moving targets. Similar nonsignificant effects were noted at higher target speeds. Ketamine-induced abnormalities were similar to those observed in relatives of schizophrenia patients under drug-free conditions. CONCLUSIONS: These results suggest that neurotransmission mediated by N-methyl-d-aspartate (NMDA) is involved in eye-tracking abnormalities. The generation of disruptive leading saccades during smooth pursuit is thought to be mediated by frontal-thalamic-cerebellar circuitry. Evidence that the locus of this and other ketamine-induced smooth-pursuit eye-movement deficits involves NMDA receptor functioning in the cerebellum is suggested.
The behavioral and pharmacological effects of the noncompetitive N-methyl-d-aspartate (NMDA) receptor antagonist ketamine have been used to study important aspects of psychotomimetic action in humans (1). Several lines of evidence support the use of ketamine as a pharmacological model of schizophrenia. Schizophrenia patients administered subanesthetic doses of ketamine experience a brief exacerbation of positive, cognitive, and possibly negative symptoms (2, 3). Ketamine also appears to provoke psychotic symptoms specific to a patient’s disease history (2). Imaging studies (4–8) have shown that ketamine changes the neuronal activity in areas thought to be involved in the pathophysiology of schizophrenia, including the medial frontal and anterior cingulate cortex, the hippocampus, and the cerebellum. Other studies have shown that ketamine causes schizophrenia-like positive, negative, and cognitive symptoms in normal healthy volunteers (9–14), including deficits in sensory processing (15) and eye-tracking performance (16, 17). Together, these data suggest that glutamatergic neurotransmission mediated by the NMDA receptor is involved in the pathophysiology of schizophrenia (18).
The presence of eye-tracking abnormalities among schizophrenia patients and their biological relatives has been reported by numerous investigators examining smooth-pursuit and saccadic eye-movement measures, as well as measures of motion perception (e.g., references 19–23). An association between eye-tracking abnormalities and NMDA receptor antagonism is important because it suggests what neurophysiological mechanisms are related to eye-tracking abnormalities. Despite numerous studies of eye tracking in patients with schizophrenia, it remains unclear what brain regions and receptor systems are involved. There is strong evidence that eye-tracking abnormalities are related to genetic risk for schizophrenia (24, 25); thus, a relationship between eye tracking and NMDA antagonism may also help us understand the biological underpinnings of disease vulnerability.
Many of the eye-tracking studies of schizophrenia patients have used global performance measures, such as root mean square error or pursuit gain. Although important in establishing the presence of disease-related abnormalities, these measures have been less informative with regard to the specific nature and neuronal localization of the deficit(s) (26). Several investigators have attempted to measure specific components of the smooth-pursuit response with encouraging results. Thaker et al. (22, 27) used target masking, a procedure in which gain is measured during brief periods of target extinction, to assess the extraretinal component of the smooth-pursuit response. On the basis of these experiments, they argued that poor eye tracking may be explained in part by an inability to use previous target motion information to produce predictive eye movements during pursuit. A predictive pursuit deficit would implicate several brain regions, including the medial-superior-temporal and posterior-parietal cortex and frontal eye fields (28–30).
Another specific aspect of the smooth-pursuit response that has received recent attention involves the appearance of disruptive rapid eye movements ahead of the target. Large-amplitude (>4°) eye movements of this type are called “anticipatory saccades.” Several investigators have found that patients produce more anticipatory saccades during pursuit than healthy comparison subjects (31–33). Studies by Ross et al. (21, 34–36) and others (37) have suggested that the presence of anticipatory saccadic eye movements is related to schizophrenia vulnerability. Patient and family differences appear particularly robust when small-amplitude (<4°) anticipatory saccades (also called leading saccades) are included (38). For example, Ross et al. (21) reported that the combined presence of anticipatory and leading saccades was the only eye-tracking measure to discriminate likely genetic carriers from familial and nonfamilial healthy comparison subjects. Abnormalities in leading-saccadic eye movements implicate a neuronal pathway from frontal eye fields to the cerebellum (21, 39–41). Of interest, both predictive pursuit and leading-saccade measures appear to improve the identification of likely genetic carriers compared with global measures (37).
The role of NMDA receptors in producing eye-tracking abnormalities is not well understood. However, many of the brain regions implicated in these abnormalities show changes in regional cerebral blood flow (rCBF) during ketamine administration. Radant et al. (16) found that ketamine decreased closed-loop pursuit gain and increased the number of catch-up saccades in normal healthy volunteers. Weiler et al. (17) found that ketamine decreased eye-movement acceleration during the initiation of smooth pursuit and decreased closed-loop pursuit gain. Of interest, ketamine did not affect predictive pursuit gain during target masking.
The effects of ketamine on anticipatory/leading saccades have not been examined. In light of recent reports suggesting that leading-saccade measures mark disease risk, we reanalyzed the data from Weiler et al. (17) to obtain leading-saccade data. On the basis of overlapping patterns of neuronal activation (e.g., in the cerebellum and prefrontal areas) observed during ketamine infusion (5–7) and during eye-tracking imaging (39), we hypothesized that ketamine would increase the frequency of leading saccades. Ketamine-induced changes would suggest a possible link between NMDA receptor functioning, neurophysiological deficit, and disease vulnerability.
Method
Clinical Assessments
Participants were recruited from the community through newspaper advertisements. All subjects gave written informed consent in accordance with guidelines from the institutional review board of the University of Maryland. Participants were screened for axis I and axis II disorders by using the Structured Clinical Interview for DSM-III-R, the Structured Clinical Interview for DSM-III-R Personality Disorders (42), and the Chapman Scales for Perceptual Aberration (43) with previously published methods (17, 22). Subjects underwent a medical history, a physical examination, and laboratory tests, including a drug screen. Participants with current or lifetime axis I or II disorders (including substance abuse), neurological disorders, a positive drug screen, or medical conditions that would render ketamine infusion unsafe were excluded from the study. Subjects were taking no medications at the time of testing.
The study participants were 10 men and two women. Their mean age was 34 years (SD=7). The relationship between age and eye-tracking performance is nonlinear, with significant deterioration appearing only after the age of about 50 (44). Mean socioeconomic status, measured with the Hollingshead-Redlich Scale (45), was 2.7 (range=1–5; higher values indicate lower socioeconomic status). All participants had previous exposure to ketamine in a laboratory setting. The average number of days between ketamine infusions was 159 (range=42–410). No adverse reactions to ketamine were observed, and all participants were able to complete the study protocol.
Laboratory Procedures
The ramp-mask-ramp task used in the present experiment has been described previously (17, 22). A foveal-petal step ramp was presented, followed by target motion in a horizontal plane, back and forth, at a constant velocity. After approximately two to three sweeps across the monitor, the target was unpredictably masked for 500 msec. Analysis of eye movements during the mask had been reported previously (17). Here, we focused on the anticipatory/leading-saccadic eye movements occurring during visible target motion. Target presentations were performed in blocks of 12 trials (six at 9.4°/second and six at 18.7°/second). Each trial consisted of four to five sweeps and one mask. The order of the trials within each block was randomly chosen. Each block of trials lasted approximately 3.5 minutes.
On each of 2 study days, the subjects had an intravenous catheter placed with a saline drip. Pulse rate and oxygen saturation were continuously monitored. After several baseline eye-tracking blocks, participants were given a ketamine (0.1 mg/kg) or placebo bolus injection over 1 minute in a double-blind fashion. Subjects were then tested on the remaining blocks. After the task, symptoms were assessed with the Brief Psychiatric Rating Scale (BPRS). Participants were randomly chosen such that six received ketamine and six received placebo on the first study day.
The dose of 0.1 mg/kg was chosen on the basis of previous dose-ranging studies. At this dose, ketamine induces mild psychotic symptoms (e.g., visual and bodily distortions), a mild blunting of affect, and greater emotional withdrawal (14). No significant blood pressure or pulse changes are noted, and no optical nystagmus is observed. Peak behavioral effects at this dose occur approximately 3 minutes after injection and last approximately 10–20 minutes. Although blood plasma levels were not obtained, previous analyses have shown that levels remain high up to 10 minutes postinfusion. Lahti et al. (14) reported mean ketamine serum levels of 110.1 ng/ml (SD=21.4) after 10 minutes and 72.5 ng/ml (SD=21.4) after 20 minutes at a dose of 0.3 mg/kg. In order to ensure that eye-tracking performance was assessed during the period of peak behavioral effects, the eye-tracking block performed immediately after the bolus injection was analyzed.
Oculomotor Data Acquisition
Eye-movement data were obtained by using infrared oculography (with a 333-Hz sampling rate and a 4-msec time constant). Data were digitized with a 16-bit analogue-to-digital converter. Digital data were filtered offline with a low-pass filter (cutoff level=75 Hz). Eye movements were analyzed with investigators blind to drug condition with interactive software. Saccades were identified by computer algorithm on the basis of velocity (>35°/second) and acceleration (>600°/second2) criteria and verified by visual inspection. Eye blinks were identified on the basis of characteristic morphology and removed (intraclass correlation coefficient [ICC] reliabilities were above 0.95).
Saccades were identified with computer algorithm and verified by visual inspection (ICC=0.95). Calkins et al. (46) have shown that eye blinks can be misidentified as anticipatory saccades when infrared oculography is used. However, they suggested that the identification of anticipatory saccades of 1°–4° in amplitude is less vulnerable to misclassification. In the present study, no amplitude criteria were employed in classifying anticipatory/leading saccades. Hereafter, we refer to both types of saccades as “leading.” Saccades were classified as “leading” if they 1) occurred in the direction of target motion, 2) either began and ended ahead of the target or if they began behind the target and jumped to a position ahead of the target, resulting in a position error equal to or greater than the original position error, or 3) were followed by at least a 50-msec period of eye velocity at 50% of target velocity for the 18.7°/second targets and 75% of target velocity for the 9.4°/second targets. Study criteria, based on amplitude, position error, and postsaccadic slowing, came from Ross et al. (44) and others (32, 39, 47). Across all conditions, a total of 730 leading saccades were identified, with a mean amplitude of 2.01° (SD=1.4). This falls within the amplitude range shown by Ross et al. (44) to maximally discriminate patients from healthy comparison subjects and the range suggested by Calkins et al. (46) to be less vulnerable to misclassification.
Three saccadic measures were assessed as follows: 1) The number of leading saccades occurring during 9.4°/second and 18.7°/second target trials were totaled for each block. 2) The ratio of time spent in leading saccades to the time spent in pursuit of the target was calculated. This is similar to the percentage of total distance owing to leading saccades, used by Ross et al. (38), and is designed to assess what percentage of visual tracking is accomplished through leading-saccadic eye movements. 3) The ratio of time spent in leading saccades to the time spent in all saccades was calculated. The ratio of time in leading to total saccades was used to evaluate the change in leading saccades relative to changes in saccadic eye movements in general. This measure is particularly important for providing evidence that leading-saccadic eye movements represent a biologically distinct schizophrenia marker.
Statistical Analyses
Preliminary analyses showed no effects of treatment order or gender. Therefore, the data were collapsed across these factors. For each participant, data were collapsed across trials to obtain mean values. The age of the subjects was not correlated with leading-saccade values or changes in leading-saccade values across treatment, phase, or target velocity conditions. The distributions for each leading-saccade measure across treatment and target velocity conditions were examined. The data approximated a normal distribution (skewness/standard error ≥2.00). Separate repeated-measures analyses of variance (ANOVAs) were performed for 9.4°/second-target and 18.7°/second-target velocities. Phase (baseline or peak [i.e., the block immediately following the infusion]) and treatment (placebo or ketamine) were entered as within-subjects factors. Analyses of simple effects were used to probe significant interactions (48). Means, standard deviations, and effect sizes are presented in Table 1.
Results
ANOVAs yielded statistically significant phase-by-treatment interactions for the number of leading saccades (F=16.54, df=1, 11, p=0.002), the ratio of time in leading saccades to time in pursuit (F=10.70, df=1, 11, p=0.007), and the ratio of time in leading saccades to time in total saccades (F=7.27, df=1, 11, p=0.02) for 9.4°/second target presentations. Simple-effects analyses showed significant increases in these measures from baseline to peak in the ketamine condition (p<0.001)—indicating an increase in the time spent off target. No significant changes occurred during the placebo condition on any measure.
The phase-by-treatment interaction for the number of leading saccades in the 18.7°/second condition was nearly significant (F=4.13, df=1, 11, p<0.07). The pattern of differences was similar, although less dramatic, in comparison to that reported for the slower target velocity. Analysis of simple effects showed that there was a significant increase from baseline to peak values for patients receiving ketamine (p=0.01); however, baseline to peak changes in patients receiving placebo were also significant (p<0.01). Phase-by-treatment interactions for the ratio of time in leading saccades to time in pursuit and the ratio of time in leading saccades to time in total saccades were not statistically significant at the 18.7°/second velocity (F=1.74, df=1, 11, p=0.21; F<1.00, df=1, 11, p=0.99, respectively). Both measures were significantly higher at peak compared to their values at baseline (i.e., main effects of phase collapsed across treatment conditions: F=17.04, df=1, 11, p=0.002; F=12.07, df=1, 11, p=0.005, respectively). The ratio of time in leading saccades to time in pursuit was nearly significant in patients receiving ketamine than in patients receiving placebo (i.e., main effect of treatment condition collapsed across phase: F=3.88, df=1, 11, p<0.08).
As reported previously (17), total BPRS scores showed a modest increase from baseline (mean=18.36, SD=0.50) to ketamine infusion (mean=20.00, SD=2.46), reflecting mild increases in score on the withdrawal and thought disorder subscales. No significant correlations were found between ketamine-induced changes in BPRS score and leading-saccade measures (correlations ranged from –0.01 to 0.46; most were <0.25). This is consistent with what we observed for patient BPRS scores and leading-saccade measures (unpublished data).
Discussion
Our results support the hypothesis that ketamine increases leading-saccadic eye movements during smooth pursuit. Because leading saccades move the eyes away from the target at a point in which initial position error is small, their presence is considered disruptive and indicative of poor performance. All three measures used in this study were significantly greater with use of ketamine in the 9.4°/second target condition. Only the number of leading saccades showed a similar but nonsignificant interaction in the 18.7°/second target condition. The ratio of time in leading saccades to time in pursuit and the ratio of time in leading saccades to total time in saccades showed a main effect of phase; the ratio of time in leading saccades to time in pursuit showed a nearly significant effect of ketamine. The effect of phase, in the absence of significant phase-by-treatment interactions, suggests that at higher target speeds, the difficulty of the task (a function of the target speed) may interact with fatigue (a function of repeated target presentations) to mask specific ketamine effects.
A robust effect of ketamine was observed in the slower target condition. This effect was not caused by an overall increase in saccadic frequency, since changes in the ratio of time in leading saccades to total time in saccades reflect a change in leading saccades above and beyond changes in overall saccadic output. The specificity of this pharmacological effect suggests that leading-saccadic eye movements are a specific marker of schizophrenia risk and supports the conclusions of Ross et al. (21, 34, 35). These results contrast with those of Radant et al. (16), who found no effect of ketamine on anticipatory saccades. Several factors may account for this discrepancy. 1) Radant et al. administered a dose sufficient to cause nystagmus, which makes the identification of leading saccades more difficult. 2) Consistent with the definitional criteria of leading saccades used by Ross et al., we included small (<4°) leading saccades. Traditionally, only saccades larger than 5° have been considered relevant. 3) Radant et al. included 20°/second targets. On the basis of our data, the inclusion of the higher target velocity may have masked the effects of ketamine.
Evidence from neuroimaging (39) and microstimulation studies (41, 49) suggests that cerebellar circuitry is involved in integrating and coordinating smooth-pursuit and saccadic eye-movement information from the frontal cortex (40, 50) via a frontal-thalamic-cerebellar circuit. Ross et al. (21) argued that abnormalities in this circuit may underlie schizophrenia-related eye-tracking abnormalities. NMDA receptors are present on cells throughout the cortex, including the frontal/prefrontal cortex and the cerebellum (18, 51, 52), where they could play a functional role in eye-tracking abnormalities. Ketamine is a noncompetitive antagonist of the NMDA receptor in these regions; thus, data from the present study are consistent with a model of eye-tracking abnormality mediated, in part, by NMDA receptor functioning within the frontal-thalamic-cerebellar circuit.
Where in this putative circuit an NMDA-induced abnormality might exist is difficult to infer. However, the absence of ketamine effects on predictive pursuit (17) suggests that the processing of extraretinal motion signals by superior mediotemporal and posterior-parietal cortical regions is spared. Similarly, frontal eye fields are likely unaffected because they mediate integration of extraretinal motion information into an ocular motor command. Also, greater rCBF in frontal areas observed during ketamine positron emission tomography imaging is more prominent in medial-frontal anterior cingulate areas than in the lateral frontal areas that include the frontal eye fields (8). In contrast, NMDA antagonism by ketamine is known to potently decrease neuronal activity in the cerebellum (8, 53), an action that can explain the observed deficits in pursuit initiation (17) and pursuit maintenance (16, 17), as well as increases in disruptive leading saccades during smooth pursuit (54–56). Several investigators have noted functional cerebellar abnormalities in patients with schizophrenia (57–59). Thus, it is possible that some of the eye-tracking deficits associated with schizophrenia risk and seen during ketamine challenge are mediated by shared cerebellar pathophysiology. This conclusion must be tempered by the possibility that changes in eye-tracking performance may be mediated through other receptor and neurotransmitter systems known to be effected by ketamine (1, 60, 61).
Given the ability of leading-saccade measures to identify schizophrenic patients and their relatives (21, 35, 37), ketamine-induced changes in leading saccades may lend insight into the possible neurophysiological underpinnings of disease risk. In this context, it would be informative to compare the eye-tracking performance of normal healthy volunteers during ketamine infusion to that of at-risk relatives of schizophrenia patients in the absence of ketamine. Similar values would suggest that the mechanisms leading to saccadic disturbance in the relatives of schizophrenia patients are similar to those operating in normal comparison subjects under conditions of NMDA antagonism. Some preliminary data are available from our laboratory to explore this question and are presented in Figure 1. Of interest, the values for the normal healthy volunteers receiving ketamine are similar to those of a group of first-degree relatives with schizophrenia spectrum symptoms, taken from Avila et al. (37). Interpretation of these data must be tempered by the possibility that normal values would change with different doses of ketamine. Nonetheless, this pattern of results, in conjunction with the neuroanatomical and neurofunctional evidence reviewed previously, raises the possibility that one of the neurophysiological mechanisms underlying genetic risk and marked by saccadic abnormalities involves NMDA-mediated neurotransmission in the cerebellum.
![]() |
Presented in part at the 31st annual meeting of the Society for Neuroscience, San Diego, Calif., Nov. 15–20, 2001. Received Dec. 3, 2001; revision received April 30, 2002; accepted May 1, 2002. From the Maryland Psychiatric Research Center. Address reprint requests to Mr. Avila, Maryland Psychiatric Research Center, P.O. Box 21247, Baltimore, MD 21228; [email protected] (e-mail). Supported by NIMH grants MH-49826 and MH-40279 and by National Institute on Drug Abuse grant DA-09483. The authors thank Heather Langley and Lisa Cooper for scoring the eye-movement data.
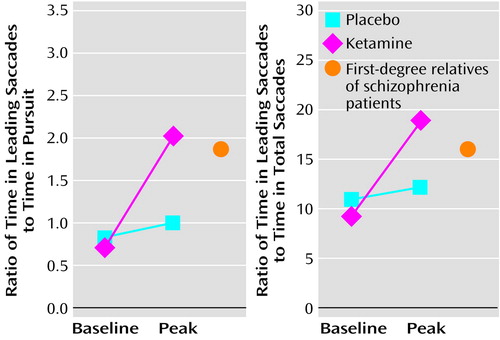
Figure 1. Eye-Tracking Performance in 12 Normal Healthy Volunteers Who Received Bolus Injections of Ketamine and Placebo and in 26 First-Degree Relatives of Schizophrenia Patients With Spectrum Personality Symptomsa
aThe targets were presented 9.4°/second. The data on first-degree relatives of schizophrenia patients with spectrum personality symptoms were presented by Avila et al. (37). Analysis of variance was used to compare the normal subjects after ketamine to the first-degree relatives. These values were not significantly different for the ratio of leading saccades to pursuit response time (F=0.67, df=1, 36, p>0.05) or for the ratio of leading to total saccade time (F=0.77, df=1, 36, p>0.05). The baseline values for the first-degree relatives were significantly higher than the baseline values of the normal subjects for the ratio of leading saccades to pursuit response time (F=5.45, df=1, 36, p<0.05) and for the ratio of leading to total saccade time (F=5.06, df=1, 36, p<0.05).
1. Jentsch JD, Roth RH: The neuropsychopharmacology of phencyclidine: from NMDA receptor hypofunction to the dopamine hypothesis of schizophrenia. Neuropsychopharmacology 1999; 20:201-225Crossref, Medline, Google Scholar
2. Lahti AC, Koffel B, Laporte D, Tamminga CA: Sub-anesthetic doses of ketamine stimulate psychosis in schizophrenia. Neuropsychopharmacology 1995; 13:9-19Crossref, Medline, Google Scholar
3. Malhotra AK, Pinals DA, Adler CM, Elman I, Clifton A, Pickar D, Breier A: Ketamine-induced exacerbation of psychotic symptoms and cognitive impairment in neuroleptic-free schizophrenics. Neuropsychopharmacology 1997; 17:141-150Crossref, Medline, Google Scholar
4. Tamminga CA, Thaker GK, Buchanan R, Kirkpatrick B, Alphs LD, Chase TN, Carpenter WT: Limbic system abnormalities identified in schizophrenia using positron emission tomography with fluorodeoxyglucose and neocortical alterations with deficit syndrome. Arch Gen Psychiatry 1992; 49:522-530Crossref, Medline, Google Scholar
5. Lahti AC, Holcomb HH, Medoff DR, Tamminga CA: Ketamine activates psychosis and alters limbic blood flow in schizophrenia. Neuroreport 1995; 6:869-872Crossref, Medline, Google Scholar
6. Breier A, Malhotra AK, Pinals DA, Weisenfeld NI, Pickar D: Association of ketamine-induced psychosis with focal activation of the prefrontal cortex in healthy volunteers. Am J Psychiatry 1997; 154:805-811Link, Google Scholar
7. Vollenweider FX, Leenders KL, Scharfetter C, Antonini A, Maguire P, Missimer J, Angst J: Metabolic hyper-frontality and psychopathology in the ketamine model of psychosis using positron emission tomography (PET) and [18F]fluorodeoxyglucose. Eur Neuropsychopharmacol 1997; 7:9-24Crossref, Medline, Google Scholar
8. Holcomb HH, Lahti AC, Medoff DR, Weiler MA, Tamminga CA: Sequential regional cerebral blood flow brain scans using PET with H215O demonstrate ketamine actions in CNS dynamically. Neuropsychopharmacology 2001; 25:165-172Crossref, Medline, Google Scholar
9. Krystal JH, Karper LP, Seibyl JP, Freeman GK, Delaney R, Bremner JD, Heninger GR, Bowers MB Jr, Charney DS: Subanesthetic effects of the noncompetitive NMDA antagonist, ketamine, in humans: psychotomimetic, perceptual, cognitive, and neuroendocrine responses. Arch Gen Psychiatry 1994; 51:199-214Crossref, Medline, Google Scholar
10. Malhotra AK, Pinals DA, Weingartner H, Sirocco K, Missar DC, Pickar D, Breier A: NMDA receptor function and human cognition: the effects of ketamine in healthy volunteers. Neuropsychopharmacology 1996; 14:301-307Crossref, Medline, Google Scholar
11. Newcomer JW, Farber NB, Jevtovic-Todorovic V, Selke G, Melson AK, Hershey T, Craft S, Olney JW: Ketamine-induced NMDA receptor hypofunction as a model of memory impairment and psychosis. Neuropsychopharmacology 1999; 20:106-118Crossref, Medline, Google Scholar
12. Adler CM, Goldberg TE, Malhotra AK, Pickar D, Breier A: Effects of ketamine on thought disorder, working memory, and semantic memory in healthy volunteers. Biol Psychiatry 1998; 43:811-816Crossref, Medline, Google Scholar
13. Adler CM, Malhotra AK, Elman I, Goldberg T, Egan M, Pickar D, Breier A: Comparison of ketamine-induced thought disorder in healthy volunteers and thought disorder in schizophrenia. Am J Psychiatry 1999; 156:1646-1649Link, Google Scholar
14. Lahti AC, Weiler MA, Michaelidis T, Parwani A, Tamminga CA: Effects of ketamine in normal and schizophrenic volunteers. Neuropsychopharmacology 2001; 25:455-467Crossref, Medline, Google Scholar
15. Oranje B, van Berkel BNM, Kemner C, van Ree JM, Kahn RS, Verbaten MN: The effects of a sub-anesthetic dose of ketamine on human selective attention. Neuropsychopharmacology 2000; 22:293-302Crossref, Medline, Google Scholar
16. Radant AD, Bowdle AT, Cowley DS, Kharasch ED, Roy-Byrne PP: Does ketamine-mediated N-methyl-d-aspartate receptor antagonism cause schizophrenia-like oculomotor abnormalities? Neuropsychopharmacology 1998; 19:434-444Crossref, Medline, Google Scholar
17. Weiler MA, Thaker GK, Lahti AC, Tamminga CA: Ketamine effects on eye movements. Neuropsychopharmacology 2000; 23:645-653Crossref, Medline, Google Scholar
18. Tamminga CA: Schizophrenia and glutamatergic transmission. Crit Rev Neurobiol 1998; 12:21-36Crossref, Medline, Google Scholar
19. Levy DL, Holzman SM, Mendell NR: Eye tracking dysfunction and schizophrenia: a critical perspective. Schizophr Bull 1993; 19:462-501Crossref, Google Scholar
20. Clementz BA, McDowell JE, Zisook S: Saccadic system functioning among schizophrenia patients and their first-degree relatives. J Abnorm Psychol 1994; 103:277-287Crossref, Medline, Google Scholar
21. Ross RG, Olincy A, Harris JG, Radant A, Adler LE, Freedman R: Anticipatory saccades during smooth pursuit eye movements and familial transmission of schizophrenia. Biol Psychiatry 1998; 44:690-697Crossref, Medline, Google Scholar
22. Thaker GK, Ross DE, Cassady S, Adami HM, Laporte D, Medoff DR, Lahti A: Smooth pursuit eye movements to extraretinal motion signals. Arch Gen Psychiatry 1998; 55:830-836Crossref, Medline, Google Scholar
23. Chen Y, Nakayama K, Levy DL, Matthysse S, Holzman PS: Psychophysical isolation of a motion-processing deficit in schizophrenics and their relatives and its association with impaired smooth pursuit. Proc Natl Acad Sci USA 1999; 96:4724-4729Crossref, Medline, Google Scholar
24. Holzman PS: On the trail of the genetics and pathophysiology of schizophrenia. Psychiatry 1996; 59:117-126Crossref, Medline, Google Scholar
25. Calkins ME, Iacono WG: Eye movement dysfunction in schizophrenia: a heritable characteristic for enhancing phenotype definition. Am J Med Genet 2000; 97:72-76Crossref, Medline, Google Scholar
26. Abel LA, Ziegler AS: Smooth pursuit eye movements in schizophrenics—what constitutes quantitative assessment? Biol Psychiatry 1988; 24:747-761Crossref, Medline, Google Scholar
27. Thaker GK, Ross DE, Buchanan RW, Adami HM, Medoff DR: Smooth pursuit eye movements to extraretinal motion signals: deficits in patients with schizophrenia. Psychiatry Res 1999; 88:209-219Crossref, Medline, Google Scholar
28. Newsome WT, Wurtz RH, Komatsu H: Relation of cortical areas MT and MST to pursuit eye movements, II: differentiation of retinal from extra-retinal inputs. J Neurophysiol 1988; 60:604-620Crossref, Medline, Google Scholar
29. Boussaoud D, Ungerleider LG, Desimone R: Pathways for motion analysis: cortical connections of the medial superior temporal and fundus of the superior temporal visual areas in the macaque. J Comp Neurol 1990; 296:462-495Crossref, Medline, Google Scholar
30. Assad JA, Maunsell JH: Neuronal correlates of inferred motion in primate posterior parietal cortex. Nature 1995; 373:518-521Crossref, Medline, Google Scholar
31. Whicker L, Abel LA, Dell’Osso LF: Smooth pursuit eye movements in the parents of schizophrenics. Neuroophthalmology 1985; 5:1-8Crossref, Google Scholar
32. Rosenberg DR, Sweeney JA, Squires-Wheeler E, Keshavan MS, Cornblatt BA, Erlenmeyer-Kimling L: Eye-tracking dysfunction in offspring from the New York High-Risk Project: diagnostic specificity and the role of attention. Psychiatry Res 1997; 66:121-130Crossref, Medline, Google Scholar
33. Levy DL, Lajonchere CM, Dorogusker B, Min D, Lee S, Tartaglini A, Lieberman JA, Mendell NR: Quantitative characterization of eye tracking dysfunction in schizophrenia. Schizophr Res 2000; 42:171-185Crossref, Medline, Google Scholar
34. Ross RG, Hommer D, Radant A, Roath M, Freedman R: Early expression of smooth-pursuit eye movement abnormalities in children of schizophrenic parents. J Am Acad Child Adolesc Psychiatry 1996; 35:941-949Crossref, Medline, Google Scholar
35. Ross RG, Olincy A, Harris JG, Radant A, Hawkins M, Adler LE, Freedman R: Evidence for bilineal inheritance of physiological indicators of risk in childhood-onset schizophrenia. Am J Med Genet 1999; 88:188-199Crossref, Medline, Google Scholar
36. Ross RG, Olincy A, Harris JG, Sullivan B, Radant AD: Smooth pursuit eye movements in schizophrenia and attentional dysfunction: adults with schizophrenia, ADHD, and a normal comparison group. Biol Psychiatry 2000; 48:197-203Crossref, Medline, Google Scholar
37. Avila MT, McMahon RP, Elliot AR, Thaker GK: Neurophysiological markers of vulnerability to schizophrenia: sensitivity and specificity of specific quantitative eye movement measures. J Abnorm Psychol 2002; 111:259-267Crossref, Medline, Google Scholar
38. Ross RG, Olincy A, Radant AD: Amplitude criteria and anticipatory saccades during smooth pursuit eye movements in schizophrenia. Psychophysiology 1999; 36:464-468Crossref, Medline, Google Scholar
39. Petit L, Clark VP, Ingeholm J, Haxby JV: Dissociation of saccade-related and pursuit-related activation in human frontal eye fields as revealed by fMRI. J Neurophysiol 1997; 77:3386-3390Crossref, Medline, Google Scholar
40. Van Gelder P, Lebedev S, Tsui WH: Predictive human pursuit and orbital goal of microstimulated smooth eye movements. J Neurophysiol 1995; 74:1358-1361Crossref, Medline, Google Scholar
41. Gottlieb JP, Bruce CJ, Macavoy MG: Smooth eye movements elicited by microstimulation in the primate frontal eye field. J Neurophysiol 1993; 69:786-799Crossref, Medline, Google Scholar
42. Spitzer RL, Williams JBW, Gibbon M, First MB: Structured Clinical Interview for DSM-III-R Personality Disorders (SCID-II). Washington, DC, American Psychiatric Press, 1990Google Scholar
43. Chapman LJ, Chapman JP: Scales for rating psychotic and psychotic-like experiences as continua. Schizophr Bull 1980; 6:477-489Medline, Google Scholar
44. Ross RG, Olincy A, Harris JG, Radant A, Adler LE, Compagnon N, Freedman R: The effects of age on a smooth pursuit tracking task in adults with schizophrenia and normal subjects. Biol Psychiatry 1999; 46:383-391Crossref, Medline, Google Scholar
45. Hollingshead AB, Redlich FC: Social Class and Mental Illness: A Community Study. New York, John Wiley & Sons, 1958Google Scholar
46. Calkins ME, Katsanis J, Hammer MA: The misclassification of blinks as saccades: implications for investigations of eye movement dysfunction in schizophrenia. Psychophysiology 2001; 38:761-767Crossref, Medline, Google Scholar
47. Radant AD, Homer DW: A quantitative analysis of saccades and smooth pursuit during visual pursuit tracking: a comparison of schizophrenics with normals and substance-abusing controls. Schizophr Res 1992; 6:225-235Crossref, Medline, Google Scholar
48. Levine G: A Guide to SPSS for Analysis of Variance. Hillsdale, NJ, Lawrence Erlbaum Associates, 1991Google Scholar
49. Ron S, Robinson DA: Eye movements evoked by cerebellar stimulation in the alert monkey. J Neurophysiol 1973; 36:1004-1022Crossref, Medline, Google Scholar
50. Suzuki DA, Keller EL: The role of posterior vermis of monkey cerebellum in smooth-pursuit eye movement control, II: target velocity-related Purkinje cell activity. J Neurophysiol 1988; 1:19-40Google Scholar
51. Conti F, Minelli A, Debiasi S, Melone M: Neuronal and glial localization of NMDA receptors in the cerebral cortex. Mol Neurobiol 1997; 14:1-18Crossref, Medline, Google Scholar
52. Thompson CL, Drewery DL, Atkins HD, Stephenson FA, Chazot PL: Immunohistochemical localization of N-methyl-d-aspartate receptor NR1, NR2A, NR2B, and NR2C/D subunits in the adult mammalian cerebellum. Neurosci Lett 2000; 283:85-88Crossref, Medline, Google Scholar
53. Porro CA, Biral GP, Benassi C, Cavazzuti M, Baraldi P, Lui F, Corazza R: Neural circuits underlying ketamine-induced oculomotor behavior in the rat: 2-deoxyglucose studies. Exp Brain Res 1999; 124:8-16Crossref, Medline, Google Scholar
54. Moschner C, Crawford TJ, Heide W, Trillenberg P, Kompf D, Kennard C: Deficits of smooth pursuit initiation in patients with degenerative cerebellar lesions. Brain 1999; 122:2147-2158Crossref, Medline, Google Scholar
55. Zee DS, Yamazaki A, Butler PH, Gucer G: Effects of ablation of flocculus and paraflocculus of eye movements in primates. J Neurophysiol 1981; 46:878-899Crossref, Medline, Google Scholar
56. Lisberger SG, Morris EJ, Tychsen L: Visual motion processing and sensory-motor integration for smooth pursuit eye movements. Annu Rev Neurosci 1987; 10:97-129Crossref, Medline, Google Scholar
57. Andreasen NC, O’Leary DS, Cizadlo T, Arndt S, Rezai K, Boles Ponto LL, Watkins GL, Hichwa RD: Schizophrenia and cognitive dysmetria: a positron-emission tomography study of dysfunctional prefrontal-thalamic-cerebellar circuitry. Proc Natl Acad Sci USA 1996; 93:9985-9990Crossref, Medline, Google Scholar
58. Andreasen NC, Paradiso S, O’Leary DS: “Cognitive dysmetria” as an integrative theory of schizophrenia: a dysfunction in cortical-subcortical-cerebellar circuitry? Schizophr Bull 1998; 24:203-218Crossref, Medline, Google Scholar
59. Martin P, Albers M: Cerebellum and schizophrenia: a selective review. Schizophr Bull 1995; 21:241-250Crossref, Medline, Google Scholar
60. Breier A, Su TP, Saunders RC, Carson RE, Kolachana BS, de Bartolomeis A, Weinberger DR, Weisenfeld N, Malhotra AK, Eckelman WC, Pickar D: Schizophrenia is associated with elevated amphetamine-induced synaptic dopamine concentrations: evidence from a novel positron emission tomography method. Proc Natl Acad Sci USA 1997; 94:2569-2574Crossref, Medline, Google Scholar
61. Kegeles LS, Abi-Dargham A, Zea-Ponce Y, Rodenhiser-Hill J, Mann JJ, Van Heertum RL, Cooper TB, Carlsson A, Laruelle M: Modulation of amphetamine-induced striatal dopamine release by ketamine in humans: implications for schizophrenia. Biol Psychiatry 2000; 48:627-640Crossref, Medline, Google Scholar