Expression of Excitatory Amino Acid Transporter Transcripts in the Thalamus of Subjects With Schizophrenia
Abstract
OBJECTIVE: Recent investigations of schizophrenia have targeted glutamatergic neurotransmission, since phencyclidine, an N-methyl-d-aspartate (NMDA) receptor antagonist, can induce schizophreniform psychosis. The authors previously reported alterations in thalamic NMDA receptor subunit expression in schizophrenia, consistent with the hypothesis that thalamic glutamatergic hypofunction may contribute to the pathophysiology of this illness. In this study they generalized this hypothesis to include other molecules of the glutamate synapse, specifically excitatory amino acid transporters (EAATs), whose normal expression and regulation in the thalamus may also be disrupted in subjects with schizophrenia. METHOD: In situ hybridization with riboprobes specific for the human excitatory amino acid transporter transcripts EAAT1, EAAT2, and EAAT3 was performed in discrete thalamic nuclei in persons with schizophrenia and comparison subjects. RESULTS: Higher expressions of transcripts encoding EAAT1 and EAAT2, but not EAAT3, were detected in the thalamus of subjects with schizophrenia. CONCLUSIONS: These findings support the hypothesis of glutamatergic dysfunction in schizophrenia and suggest that molecules other than glutamate receptors are abnormally expressed in glutamatergic synapses in this illness.
Recent investigations in schizophrenia have targeted glutamatergic neurotransmission in the thalamus because of both its central role in sensory processing and its rich glutamatergic connections to limbic regions of the brain (1). Several lines of evidence have implicated thalamic abnormalities in subjects with schizophrenia. Studies that used magnetic resonance imaging or unbiased volume estimation techniques have reported thalamic volume deficits in patients with schizophrenia (2–7). Other work that used positron emission tomography or single photon emission computed tomography detected abnormalities in thalamic metabolism, while other studies have reported deficits in the number of thalamic neurons and glia in patients with schizophrenia (5, 8–14). The reported deficits in thalamic volume, metabolism, and cell number in patients with schizophrenia are consistent with other work that has demonstrated that persons with schizophrenia have deficits in recalling complex narrative material, which suggests an abnormality in cortico-thalamic-cerebellar connectivity (11, 12). While these data suggest the thalamus as a candidate region for pathophysiology in schizophrenia, they do not indicate which neurochemical substrates are associated with these abnormalities. The glutamatergic system is a likely candidate, since pharmacological evidence has implicated glutamatergic dysfunction in schizophrenia.
Glutamate receptors are implicated in the pathophysiology of schizophrenia in part because phencyclidine (PCP) induces schizophreniform psychosis (15–19). PCP binds to the intrachannel site of the N-methyl-d-aspartate (NMDA) receptor, blocking normal receptor activity (20, 21). Thus, abnormalities in thalamic glutamatergic neurotransmission may contribute to the pathophysiology of schizophrenia. Previous work in our laboratory has detected lower expression of some NMDA receptor subunits and binding sites in the thalamus of subjects with schizophrenia (22). Striking alterations in AMPA and kainate subunit mRNA and binding site expression were not detected (22), and there were no significant changes in the expression of transcripts encoding the metabotropic glutamate receptors in the thalamus in schizophrenia (23). These results are consistent with the hypothesis that NMDA receptor hypoactivity may contribute to psychopathology in schizophrenia. In addition to the NMDA receptor, however, other synaptic elements, such as excitatory amino acid transporters (EAATs), may directly affect glutamatergic neurotransmission in schizophrenia.
Recently, a novel family of excitatory amino acid transporters was cloned and characterized (24). This family of sodium-dependent glutamate/aspartate transporters includes five members (EAAT1–EAAT5) and is structurally similar to the neutral amino acid transporters ASCT1 and ASCT2 but is unrelated to other neurotransmitter transporters (25, 26). Structural studies have predicted that these transporters possess six to 10 transmembrane domains, which may form an aqueous transmembrane pore (27). The transporter is reversible when the sodium gradient is altered, such as during a seizure (28). It is of interest that EAAT4 and EAAT5 appear to have the unique property of gating chloride ions (29, 30). The transcripts have specific patterns of cellular localization: EAAT1 and EAAT2 have been localized to astroglia, whereas EAAT3 and EAAT4 are localized to neurons (31–37).
The best studied of the glutamate transporters, EAAT2 (called GLT-1 in the rodent), accounts for approximately 90% of rodent forebrain glutamate reuptake (38, 39). Expression of EAAT2 protein and mRNA has been observed throughout the human brain but is highest in the forebrain (31, 32). Astrocytes have been identified as the predominant cell type expressing EAAT2 protein by immunohistochemical staining (33–35). GLT-1 knockout mice exhibit hippocampal neurodegeneration and develop lethal seizures, emphasizing the physiologic importance of homeostatic regulation of glutamate levels (38, 39).
Similar to EAAT2, EAAT3 (called EAAC1 in the rodent) protein expression in the human brain is detected in the frontal cortex and the hippocampus (31). Postmortem human EAAT3 mRNA localization has not been evaluated. EAAT3 is localized to both post- and presynaptic neuronal soma and contributes approximately 40% of hippocampal glutamate transport (34). In contrast, levels of EAAT1 (called GLAST in the rodent) and EAAT4 protein expression in rodent CNS are highest in the cerebellum, in the Bergmann glia and Purkinje cell types, respectively (31, 36, 37). Human studies of EAAT1 protein expression indicate high levels in the frontal cortex, while EAAT1 and EAAT4 mRNA levels have not been extensively examined in humans. CNS EAAT5 mRNA expression is limited to the retina (29).
Subtle functional differences between the glutamate transporters illustrate the complexity of the glutamate synapse. For example, EAAT2, but not EAAT1, is inhibited by dihydrokainate, suggesting that anatomic or circuit-dependent differences in glutamate transporter pharmacology may reflect differences in the functional nature of a given glutamate synapse or circuit (40, 41). The variability in effects of transporter antisense or knockout experiments is consistent with this notion. GLT-1 (EAAT2) knockout animals develop lethal seizures, while GLAST (EAAT1) knockout animals have subtler defects, including difficulties with coordination (38, 39, 42). It is not surprising that alterations in excitatory amino acid transporter expression have been reported in schizophrenia, Huntington’s disease, and amyotrophic lateral sclerosis (43–48). It is interesting to note that variable splicing of EAAT2 in amyotrophic lateral sclerosis subjects has been detected, implicating alterations in mRNA processing as a possible etiology for abnormalities in excitatory amino acid transporter expression in human diseases (45). Thus, we hypothesized that in schizophrenia, an abnormality in excitatory amino acid transporter expression may contribute to glutamatergic dysfunction. The aim of this study was to investigate thalamic expression of members of the family of excitatory amino acid transporters (EAAT1, EAAT2, EAAT3, and EAAT4) in the brain of subjects with schizophrenia.
Method
Subjects
Twelve subjects with schizophrenia (five women and seven men; mean age=70 years [SD=8], mean postmortem interval=420 minutes [SD=328]) and eight individuals with no psychiatric illness (six women and two men; mean age=77 years [SD=14], mean postmortem interval=613 minutes [SD=425]) from the Mount Sinai Medical Center Brain Bank were studied. The schizophrenic subjects in the present study are the same as those used in our previous reports on glutamate receptor expression in the thalamus in schizophrenia (22, 23). Subjects were classified as having schizophrenia if 1) the presence of schizophrenic symptoms could be documented before age 40; 2) the medical records contained evidence of psychotic symptoms and at least 10 years of psychiatric hospitalization with a diagnosis of schizophrenia; 3) the DSM-III-R diagnosis of schizophrenia was agreed upon by two experienced clinicians; and 4) neuropathological examination did not reveal Alzheimer’s disease or other degenerative disorders. Subjects with a history of alcoholism or substance abuse were excluded from this cohort. Neither age (t=1.6, df=18, p>0.10), postmortem interval (t=1.1, df=18, p=0.30), nor sex distribution (χ2=2.15, df=1, p>0.10) were significantly different between the two groups. At the time of death of the persons with schizophrenia, six were receiving antipsychotics, five had a mean drug-free period of 5.8 weeks (SD=3.4), and one subject had been drug free for 416 weeks.
In Situ Hybridization
Brains were obtained at autopsy, and 20-μm sections were prepared as previously described (22, 23). Expression of EAAT1, EAAT2, EAAT3, and EAAT4 mRNA was determined by using in situ hybridization. To generate subclones from which to synthesize riboprobes, we amplified unique regions of EAAT1 (National Center for Biotechnology GenBank assession number: U03504, nucleotide coding region: 526–825), EAAT2 (NM004171, 601–1026), EAAT3 (NM004170, 156–979), and EAAT4 (NM005071, 541–900) from a human cDNA brain library (EdgeBiosystems, Gaithersburg, Md.) by using polymerase chain reaction (PCR). For EAAT2, the region selected binds to all known splice variants. Amplified cDNA segments were extracted (QIAquick Gel Extraction Kit, Qiagen, Valencia, Calif.), subcloned (Zero Blunt TOPO PCR cloning kit, Invitrogen, Carlsbad, Calif.), and confirmed by nucleotide sequencing (Thermo Sequenase Radiolabeled Termination Cycle Sequencing Kit, USB, Cleveland). Riboprobes were synthesized from linearized plasmid DNA, and in situ hybridization was performed as previously described (22). Briefly, radiolabeled probes were diluted in 50% (vol:vol) formamide hybridization buffer, applied to sections, and incubated overnight at 55°C. In situ hybridization was performed with sense and antisense probes for EAAT1, EAAT2, EAAT3, and EAAT4 on serial sections of macaque thalamus to confirm probe specificity.
Data Analysis
Thalamic nuclei were identified in each section on the basis of cellular and white matter patterns, defined by cresyl violet and gold chloride staining of adjacent sections from each subject as previously described (22, 23). Subdivisions of thalamic nuclei were not clearly distinguishable in all sections for all subjects, and subdivisions were pooled for data analysis. The following nuclei were identified for each subject: anterior, dorsal medial, lateral dorsal, central medial, ventral, and reticular. In this study, “ventral” nucleus corresponds to a grouping of several nuclei of the ventral tier. Images were digitized from films and analyzed with Image 1.56 (National Institutes of Health, Bethesda, Md.). For digitized images, gray-scale values of tissue background were subtracted from values for each nucleus and converted to optical densities. Values from two sections for each subject were averaged and used for statistical analysis. Statistical analysis was performed for each probe by two-way analysis of variance, with diagnosis and nuclei as the independent variables. Post hoc analyses were by the Newman-Keuls test. We analyzed the relationship between age and excitatory amino acid transporter expression in each nucleus with a series of correlation coefficients. For all tests, alpha=0.05.
Results
Sense and antisense probes for EAAT1, EAAT2, EAAT3, and EAAT4 transcripts were tested in sections of macaque thalamus; specific labeling was only observed for sections incubated with antisense riboprobe (data not shown). EAAT4 mRNA expression was not distinguishable from background in the macaque thalamus and thus was not included in this study. EAAT1, EAAT2, and EAAT3 were expressed in all nuclei studied (Figure 1 and Table 1). As seen in Table 1, there was a main effect of diagnosis for thalamic expression of EAAT1 and EAAT2; there was no main effect of diagnosis for thalamic expression of EAAT3 (F=1.65, df=1, 108, p<0.21). We did not detect any significant diagnosis-by-thalamic nucleus interactions. Post hoc analysis revealed levels of EAAT1, EAAT2, and EAAT3 mRNA expression in the reticular nucleus and EAAT1 and EAAT3 mRNA expression in the ventral nucleus that were significantly lower than the levels of expression in the other thalamic nuclei for both the schizophrenic and comparison subjects. The largest percentage difference in EAAT mRNA expression was observed in the reticular nucleus (Figure 2). We did not detect a significant relationship between age and excitatory amino acid transporter expression in any thalamic nuclei.
Discussion
In this study, we detected significantly higher levels of EAAT1 and EAAT2 mRNA expression in the thalamus of subjects with schizophrenia, to our knowledge the first such demonstration in this illness. Significant changes in excitatory amino acid transporter expression were not specific to any of the six nuclei examined (no significant diagnosis-by-nucleus interaction), suggesting that small to moderate increases throughout all of the thalamic nuclei examined account for our finding. This result is consistent with the hypothesis that glutamatergic function in the thalamus of schizophrenic subjects is abnormal.
We have previously reported subunit- and binding site-specific deficits in ionotropic glutamate receptor expression in the limbic thalamus in schizophrenia, limited primarily to NMDA receptors (22, 23). One unifying interpretation of our current and previous data is that an abnormality of presynaptic glutamate release resulting in higher synaptic glutamate levels might stimulate a compensatory increase in glial EAAT1 and EAAT2 expression and decrease expression of postsynaptic NMDA receptors. The juxtaposition of lower NMDA receptor expression and higher excitatory amino acid transporter expression may be further explained by other putative homeostatic regulatory mechanisms (49). Cytokines, growth factors, protein kinases, phospholipases, and cyclic lipid mediators have all been implicated as factors regulating glutamate reuptake (50–55). One mechanism that may directly link NMDA receptor function with excitatory amino acid transporter expression involves arachidonic acid release. In vitro studies indicate that arachidonic acid is released following activation of NMDA receptors and attenuates glutamate uptake in neurons and glia (56–63). Such a mechanism might lead to higher levels of excitatory amino acid transporter expression if lower levels of NMDA receptor expression led to less stimulation of arachidonic acid synthesis, thereby disinhibiting excitatory amino acid transporter expression.
Other studies have demonstrated alterations in excitatory amino acid transporter expression in subjects with schizophrenia. A higher ratio of mGluR3/EAAT2 and mGluR5/EAAT2 mRNA expression was detected in the prefrontal cortex of subjects with schizophrenia (48). In this study, however, there were no significant changes in either EAAT2 or mGluR mRNA expression when not expressed as a ratio. In another study, lower expression of EAAT2, but not EAAT1 or EAAT3, mRNA was detected in the superficial and deep layers of the dorsolateral prefrontal cortex in a cohort of subjects with schizophrenia (64, 65). Such changes suggest an abnormality in a reciprocal glutamatergic corticothalamic circuit in schizophrenia.
While there are no data evaluating antipsychotic effects on excitatory amino acid transporter expression in the thalamus, the prefrontal cortex and striatum have been studied. In the striatum of rats treated for 30 days with either haloperidol or clozapine, expression of EAAT2 mRNA was lower (66). Consistent with this finding, in the striatum of rats treated for 27 weeks with haloperidol, d-[3H]aspartate uptake was significantly attenuated (67). Another study demonstrated a decrease in EAAT2 mRNA expression in the cortex of rats following 9 weeks of treatment with clozapine (68). These data suggest that antipsychotic exposure decreases EAAT expression, suggesting that our finding of elevated expression in schizophrenia is not a drug effect.
Our results demonstrate that alterations in transporter expression in schizophrenia are limited to the glial-based transporters, EAAT1 and EAAT2 (24). Since EAAT3 expression is neuronal and somatodendritic, we speculate that neuronal transporter expression is not affected in thalamic glutamatergic synapses of subjects with schizophrenia (24, 34, 49). The dramatic effects of GLAST (EAAT1) or GLT-1 (EAAT2) knockout mice, versus the relatively quiescent effects of knocking out EAAC1 (EAAT3), suggest differential functional roles for the transporters that may be reflected in the differential patterns of transporter expression (31–39, 42).
Previously, we reported changes in NMDA receptor (NR) subunit and binding site expression in the thalamus of subjects with schizophrenia (22). The specific findings of alterations in NR1 and NR2C subunit expression and binding to the polyamine modulatory site suggested a change in NMDA receptor stoichiometry, while decreases in expression of the requisite NR1 subunit suggested a deficit in NMDA receptor expression on the cell surface (22). We now extend our findings of thalamic alterations in schizophrenia to the glutamate transporter family. The finding of higher levels of EAAT1 and EAAT2 expression, taken together with deficits in NMDA receptor expression, suggest differential abnormalities in schizophrenia within families of related molecules. These results highlight the potential importance of examining different components of the glutamate synapse and directly support the notion of thalamic glutamatergic dysfunction in schizophrenia.
![]() |
Presented in part at the 30th annual meeting of the Society for Neuroscience, New Orleans, Nov. 4–9, 2000. Received Dec. 19, 2000; revision received April 20, 2001; accepted April 25, 2001. From the Department of Psychiatry/Mental Health Research Institute, University of Michigan Medical School; and the Department of Psychiatry, Mount Sinai School of Medicine, New York. Address reprint requests to Dr. Smith, Department of Psychiatry/Mental Health Research Institute, University of Michigan Medical School, 205 Zina Pitcher Place, Ann Arbor, MI 48109-0720; [email protected] (e-mail). Supported by a grant from NIMH (MH-53327) and an Independent Investigator Award from the National Alliance for Research on Schizophrenia and Depression to Dr. Meador-Woodruff; a Veterans Merit Review Award to Dr. Haroutunian; and grants from NIMH (MH-45212) and the National Institute on Aging (AG-5138, AG-2219) to Dr. Davis.
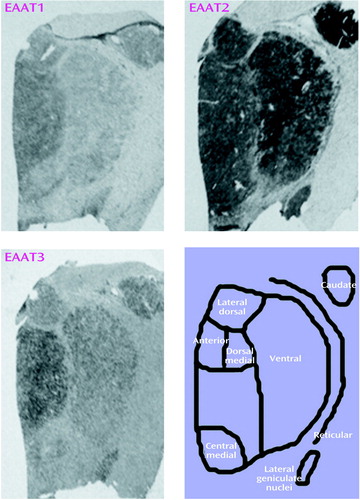
Figure 1. Expression of Excitatory Amino Acid Transporter (EAAT) Transcripts in Thalamic Nuclei of a Subject With Schizophrenia
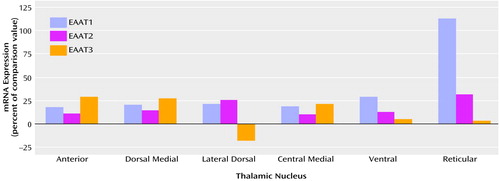
Figure 2. Expression of mRNA in Excitatory Amino Acid Transporter (EAAT) Transcripts in Thalamic Nuclei of 12 Subjects With Schizophrenia, Relative to Expression in Eight Comparison Subjects
1. Steriade M, Jones EG, McCormick DA: The Thalamus. Oxford, UK, Elsevier, 1997Google Scholar
2. Andreasen NC, Arndt S, Swayze V II, Cizadlo T, Flaum M, O’Leary D, Ehrhardt JC, Yuh WTC: Thalamic abnormalities in schizophrenia visualized through magnetic resonance image averaging. Science 1994; 266:294-298Crossref, Medline, Google Scholar
3. Pakkenberg B: The volume of the mediodorsal thalamic nucleus in treated and untreated schizophrenics. Schizophr Res 1992; 7:95-100Crossref, Medline, Google Scholar
4. Arciniegas D, Rojas DC, Teale P, Sheeder J, Sandberg E, Reite M: The thalamus and the schizophrenia phenotype: failure to replicate reduced volume. Biol Psychiatry 1999; 45:1329-1335Google Scholar
5. Buchsbaum MS, Someya T, Teng CY, Abel L, Chin S, Najafi A, Haier RJ, Wu J, Bunney WE Jr: PET and MRI of the thalamus in never-medicated patients with schizophrenia. Am J Psychiatry 1996; 153:191-199Link, Google Scholar
6. Portas CM, Goldstein JM, Shenton ME, Hokama HH, Wible CG, Fischer I, Kikinis R, Donnino R, Jolesz FA, McCarley RW: Volumetric evaluation of the thalamus in schizophrenic male patients using magnetic resonance imaging. Biol Psychiatry 1998; 43:649-659Crossref, Medline, Google Scholar
7. Staal WG, Hulshoff Pol HE, Schnack H, van der Schot AC, Kahn RS: Partial volume decrease of the thalamus in relatives of patients with schizophrenia. Am J Psychiatry 1998; 155:1784-1786Google Scholar
8. Vita A, Bressi S, Perani D, Invernizzi G, Giobbio GM, Dieci M, Garbarini M, Del Sole A, Fazio F: High-resolution SPECT study of regional cerebral blood flow in drug-free and drug-naive schizophrenic patients. Am J Psychiatry 1995; 152:876-882Link, Google Scholar
9. Buchsbaum MS, Hazlett EA: Positron emission tomography studies of abnormal glucose metabolism in schizophrenia. Schizophr Bull 1998; 24:343-364Crossref, Medline, Google Scholar
10. Hazlett EA, Buchsbaum MS, Byne W, Wei TC, Spiegel-Cohen J, Geneve C, Kinderlehrer R, Haznedar MM, Shihabuddin L, Siever LJ: Three-dimensional analysis with MRI and PET of the size, shape, and function of the thalamus in the schizophrenia spectrum. Am J Psychiatry 1999; 156:1190-1199Google Scholar
11. Andreasen NC, O’Leary DS, Cizadlo T, Arndt S, Rezai K, Boles Ponto LL, Watkins GL, Hichwa RD: Schizophrenia and cognitive dysmetria: a positron-emission tomography study of dysfunctional prefrontal-thalamic-cerebellar circuitry. Proc Natl Acad Sci USA 1996; 93:9985-9990Google Scholar
12. Andreasen NC, Paradiso S, O’Leary DS: “Cognitive dysmetria” as an integrative theory of schizophrenia: a dysfunction in cortical-subcortical-cerebellar circuitry? Schizophr Bull 1998; 24:203-218Crossref, Medline, Google Scholar
13. Young KA, Manaye KF, Liang C, Hicks PB, German DC: Reduced number of mediodorsal and anterior thalamic neurons in schizophrenia. Biol Psychiatry 2000; 47:944-953Crossref, Medline, Google Scholar
14. Pakkenberg B: Pronounced reduction of total neuron number in mediodorsal thalamic nucleus and nucleus accumbens in schizophrenics. Arch Gen Psychiatry 1990; 47:1023-1028Google Scholar
15. Javitt DC, Zukin SR: Recent advances in the phencyclidine model of schizophrenia. Am J Psychiatry 1991; 148:1301-1308Google Scholar
16. Krystal JH, Karper LP, Seibyl JP, Freeman GK, Delaney R, Bremner JD, Heninger GR, Bowers MB Jr, Charney DS: Subanesthetic effects of the noncompetitive NMDA antagonist, ketamine, in humans: psychotomimetic, perceptual, cognitive, and neuroendocrine responses. Arch Gen Psychiatry 1994; 51:199-214Crossref, Medline, Google Scholar
17. Lahti AC, Holcomb HH, Medoff DR, Tamminga CA: Ketamine activates psychosis and alters limbic blood flow in schizophrenia. Neuroreport 1995; 6:869-872Crossref, Medline, Google Scholar
18. Luby ED, Gottlieb JS, Cohen BD, Rosenbaum G, Domino EF: Model psychoses and schizophrenia. Am J Psychiatry 1962; 119:61-67Link, Google Scholar
19. Itil T, Keskiner A, Kiremitci N, Holden JM: Effect of phencyclidine in chronic schizophrenics. Can Psychiatr Assoc J 1967; 12:209-212Crossref, Medline, Google Scholar
20. Aanonsen LM, Wilcox GL: Phencyclidine selectively blocks a spinal action of N-methyl-d-aspartate in mice. Neurosci Lett 1986; 67:191-197Crossref, Medline, Google Scholar
21. Jentsch JD, Roth RH: The neuropsychopharmacology of phencyclidine: from NMDA receptor hypofunction to the dopamine hypothesis of schizophrenia. Neuropsychopharmacology 1999; 20:201-225Crossref, Medline, Google Scholar
22. Ibrahim HM, Hogg AJ, Healy DJ Jr, Haroutunian V, Davis KL, Meador-Woodruff JH: Ionotropic glutamate receptor binding and subunit mRNA expression in thalamic nuclei in schizophrenia. Am J Psychiatry 2000; 157:1811-1823Google Scholar
23. Richardson-Burns SM, Haroutunian V, Davis KL, Watson SJ, Meador-Woodruff JH: Metabotropic glutamate receptor mRNA expression in the schizophrenic thalamus. Biol Psychiatry 2000; 47:22-28Crossref, Medline, Google Scholar
24. Sims KD, Robinson MB: Expression patterns and regulation of glutamate transporters in the developing and adult nervous system. Crit Rev Neurobiol 1999; 13:169-197Crossref, Medline, Google Scholar
25. Arriza JL, Kavanaugh MP, Fairman WA, Wu YN, Murdoch GH, North RA, Amara SG: Cloning and expression of a human neutral amino acid transporter with structural similarity to the glutamate transporter gene family. J Biol Chem 1993; 268:15329-15332Google Scholar
26. Utsunomiya-Tate N, Endou H, Kanai Y: Cloning and functional characterization of a system ASC-like Na+-dependent neutral amino acid transporter. J Biol Chem 1996; 271:14883-14890Google Scholar
27. Seal RP, Amara SG: A reentrant loop domain in the glutamate carrier EAAT1 participates in substrate binding and translocation. Neuron 1998; 21:1487-1498Google Scholar
28. Del Arco A, Gonzalez-Mora JL, Armas VR, Mora F: Amphetamine increases the extracellular concentration of glutamate in striatum of the awake rat: involvement of high affinity transporter mechanisms. Neuropharmacology 1999; 38:943-954Crossref, Medline, Google Scholar
29. Arriza JL, Eliasof S, Kavanaugh MP, Amara SG: Excitatory amino acid transporter 5, a retinal glutamate transporter coupled to a chloride conductance. Proc Natl Acad Sci USA 1997; 94:4155-4160Google Scholar
30. Nagao S, Kwak S, Kanazawa I: EAAT4, a glutamate transporter with properties of a chloride channel, is predominantly localized in Purkinje cell dendrites, and forms parasagittal compartments in rat cerebellum. Neuroscience 1997; 78:929-933Crossref, Medline, Google Scholar
31. Bar-Peled O, Ben-Hur H, Biegon A, Groner Y, Dewhurst S, Furuta A, Rothstein JD: Distribution of glutamate transporter subtypes during human brain development. J Neurochem 1997; 69:2571-2580Google Scholar
32. Milton ID, Banner SJ, Ince PG, Piggott NH, Fray AE, Thatcher N, Horne CH, Shaw PJ: Expression of the glial glutamate transporter EAAT2 in the human CNS: an immunohistochemical study. Brain Res Mol Brain Res 1997; 52:17-31Crossref, Medline, Google Scholar
33. Lehre KP, Levy LM, Ottersen OP, Storm-Mathisen J, Danbolt NC: Differential expression of two glial glutamate transporters in the rat brain: quantitative and immunocytochemical observations. J Neurosci 1995; 15:1835-1853Google Scholar
34. Rothstein JD, Martin L, Levey AI, Dykes-Hoberg M, Jin L, Wu D, Nash N, Kuncl RW: Localization of neuronal and glial glutamate transporters. Neuron 1994; 13:713-725Crossref, Medline, Google Scholar
35. Furuta A, Rothstein JD, Martin LJ: Glutamate transporter protein subtypes are expressed differentially during rat CNS development. J Neurosci 1997; 17:8363-8375Google Scholar
36. Yamada K, Watanabe M, Shibata T, Tanaka K, Wada K, Inoue Y: EAAT4 is a post-synaptic glutamate transporter at Purkinje cell synapses. Neuroreport 1996; 7:2013-2017Google Scholar
37. Yamada K, Wada S, Watanabe M, Tanaka K, Wada K, Inoue Y: Changes in expression and distribution of the glutamate transporter EAAT4 in developing mouse Purkinje cells. Neurosci Res 1997; 27:191-198Crossref, Medline, Google Scholar
38. Rothstein JD, Dykes-Hoberg M, Pardo CA, Bristol LA, Jin L, Kuncl RW, Kanai Y, Hediger MA, Wang Y, Schielke JP, Welty DF: Knockout of glutamate transporters reveals a major role for astroglial transport in excitotoxicity and clearance of glutamate. Neuron 1996; 16:675-686Crossref, Medline, Google Scholar
39. Tanaka K, Watase K, Manabe T, Yamada K, Watanabe M, Takahashi K, Iwama H, Nishikawa T, Ichihara N, Kikuchi T, Okuyama S, Kawashima N, Hori S, Takimoto M, Wada K: Epilepsy and exacerbation of brain injury in mice lacking the glutamate transporter GLT-1. Science 1997; 276:1699-1702Google Scholar
40. Dunlop J, Lou Z, Zhang Y, McIlvain HB: Inducible expression and pharmacology of the human excitatory amino acid transporter 2 subtype of L-glutamate transporter. Br J Pharmacol 1999; 128:1485-1490Google Scholar
41. Robinson MB: The family of sodium-dependent glutamate transporters: a focus on the GLT-1/EAAT2 subtype. Neurochem Int 1998; 33:479-491Crossref, Medline, Google Scholar
42. Watase K, Hashimoto K, Kano M, Yamada K, Watanabe M, Inoue Y, Okuyama S, Sakagawa T, Ogawa S, Kawashima N, Hori S, Takimoto M, Wada K, Tanaka K: Motor discoordination and increased susceptibility to cerebellar injury in GLAST mutant mice. Eur J Neurosci 1998; 10:976-988Crossref, Medline, Google Scholar
43. Meyer T, Fromm A, Munch C, Schwalenstocker B, Fray AE, Ince PG, Stamm S, Gron G, Ludolph AC, Shaw PJ: The RNA of the glutamate transporter EAAT2 is variably spliced in amyotrophic lateral sclerosis and normal individuals. J Neurol Sci 1999; 170:45-50Crossref, Medline, Google Scholar
44. Meyer T, Munch C, Volkel H, Booms P, Ludolph AC: The EAAT2 (GLT-1) gene in motor neuron disease: absence of mutations in amyotrophic lateral sclerosis and a point mutation in patients with hereditary spastic paraplegia. J Neurol Neurosurg Psychiatry 1998; 65:594-596Crossref, Medline, Google Scholar
45. Lin CL, Bristol LA, Jin L, Dykes-Hoberg M, Crawford T, Clawson L, Rothstein JD: Aberrant RNA processing in a neurodegenerative disease: the cause for absent EAAT2, a glutamate transporter, in amyotrophic lateral sclerosis. Neuron 1998; 20:589-602Crossref, Medline, Google Scholar
46. Fray AE, Ince PG, Banner SJ, Milton ID, Usher PA, Cookson MR, Shaw PJ: The expression of the glial glutamate transporter protein EAAT2 in motor neuron disease: an immunohistochemical study. Eur J Neurosci 1998; 10:2481-2489Google Scholar
47. Arzberger T, Krampfl K, Leimgruber S, Weindl A: Changes of NMDA receptor subunit (NR1, NR2B) and glutamate transporter (GLT1) mRNA expression in Huntington’s disease—an in situ hybridization study. J Neuropathol Exp Neurol 1997; 56:440-454Crossref, Medline, Google Scholar
48. Ohnuma T, Augood SJ, Arai H, McKenna PJ, Emson PC: Expression of the human excitatory amino acid transporter 2 and metabotropic glutamate receptors 3 and 5 in the prefrontal cortex from normal individuals and patients with schizophrenia. Brain Res Mol Brain Res 1998; 56:207-217Crossref, Medline, Google Scholar
49. Arriza JL, Fairman WA, Wadiche JI, Murdoch GH, Kavanaugh MP, Amara SG: Functional comparisons of three glutamate transporter subtypes cloned from human motor cortex. J Neurosci 1994; 14:5559-5569Google Scholar
50. Dorandeu F, Antier D, Pernot-Marino I, Lapeyre P, Lallement G: Venom phospholipase A2-induced impairment of glutamate uptake: an indirect and nonselective effect related to phospholipid hydrolysis. J Neurosci Res 1998; 51:349-359Crossref, Medline, Google Scholar
51. Hu S, Sheng WS, Ehrlich LC, Peterson PK, Chao CC: Cytokine effects on glutamate uptake by human astrocytes. Neuroimmunomodulation 2000; 7:153-159Crossref, Medline, Google Scholar
52. Daniels KK, Vickroy TW: Reversible activation of glutamate transport in rat brain glia by protein kinase C and an okadaic acid-sensitive phosphoprotein phosphatase. Neurochem Res 1999; 24:1017-1025Google Scholar
53. Dixon JF, Hokin LE: Lithium acutely inhibits and chronically up-regulates and stabilizes glutamate uptake by presynaptic nerve endings in mouse cerebral cortex. Proc Natl Acad Sci USA 1998; 95:8363-8368Google Scholar
54. Palos TP, Zheng S, Howard BD: Wnt signaling induces GLT-1 expression in rat C6 glioma cells. J Neurochem 1999; 73:1012-1023Google Scholar
55. Gegelashvili G, Dehnes Y, Danbolt NC, Schousboe A: The high-affinity glutamate transporters GLT1, GLAST, and EAAT4 are regulated via different signalling mechanisms. Neurochem Int 2000; 37:163-170Crossref, Medline, Google Scholar
56. Viu E, Zapata A, Capdevila JL, Fossom LH, Skolnick P, Trullas R: Glycine site antagonists and partial agonists inhibit N-methyl-d-aspartate receptor-mediated [3H]arachidonic acid release in cerebellar granule cells. J Pharmacol Exp Ther 1998; 285:527-532Medline, Google Scholar
57. Manzoni C, Mennini T: Arachidonic acid inhibits 3H-glutamate uptake with different potencies in rodent central nervous system regions expressing different transporter subtypes. Pharmacol Res 1997; 35:149-151Crossref, Medline, Google Scholar
58. Lazarewicz JW, Salinska E, Wroblewski JT: NMDA receptor-mediated arachidonic acid release in neurons: role in signal transduction and pathological aspects. Adv Exp Med Biol 1992; 318:73-89Crossref, Medline, Google Scholar
59. Lazarewicz JW, Wroblewski JT, Palmer ME, Costa E: Activation of N-methyl-d-aspartate-sensitive glutamate receptors stimulates arachidonic acid release in primary cultures of cerebellar granule cells. Neuropharmacology 1988; 27:765-769Crossref, Medline, Google Scholar
60. Lazarewicz JW, Wroblewski JT, Costa E: N-Methyl-d-aspartate-sensitive glutamate receptors induce calcium-mediated arachidonic acid release in primary cultures of cerebellar granule cells. J Neurochem 1990; 55:1875-1881Google Scholar
61. Volterra A, Trotti D, Racagni G: Glutamate uptake is inhibited by arachidonic acid and oxygen radicals via two distinct and additive mechanisms. Mol Pharmacol 1994; 46:986-992Medline, Google Scholar
62. Farooqui AA, Horrocks LA: Excitatory amino acid receptors, neural membrane phospholipid metabolism and neurological disorders. Brain Res Brain Res Rev 1991; 16:171-191Crossref, Medline, Google Scholar
63. Dumuis A, Sebben M, Haynes L, Pin JP, Bockaert J: NMDA receptors activate the arachidonic acid cascade system in striatal neurons. Nature 1988; 336:68-70Crossref, Medline, Google Scholar
64. Hyde TM, Shannon Weickert C, Romanczyk TB, Eisenberg N, Shashidharan P, Herman MM, Bachus SE, Weinberger DR, Kleinman JE: Dorsolateral prefrontal cortex pathology in schizophrenia: excitatory amino acid transporters 1 and 2, in Abstracts of the 30th Annual Meeting of the Society for Neuroscience. Washington, DC, SFN, 2000Google Scholar
65. Crook JM, Shannon Weickert C, Tomaskovic-Crook E, Lin JC, Herman MM, Hyde TM, Kleinman JE: Excitatory amino acid transporter 3 mRNA and protein in DLPFC of schizophrenia. IbidGoogle Scholar
66. Schneider JS, Wade T, Lidsky TI: Chronic neuroleptic treatment alters expression of glial glutamate transporter GLT-1 mRNA in the striatum. Neuroreport 1998; 9:133-136Crossref, Medline, Google Scholar
67. De Souza IE, McBean GJ, Meredith GE: Chronic haloperidol treatment impairs glutamate transport in the rat striatum. Eur J Pharmacol 1999; 382:139-142Crossref, Medline, Google Scholar
68. Melone M, Vallejo A, Perez-Samartin A, Matute C, Cozzi A, Pellegrini-Giampietro DE, Vitellaro-Zuccarello L, Barbaresi P, Conti F: Expression and function of glutamate transporter GLT-1 are downregulated by the antipsychotic clozapine, in Abstracts of the 30th Annual Meeting of the Society for Neuroscience. Washington, DC, SFN, 2000Google Scholar