Higher Cortical and Lower Subcortical Metabolism in Detoxified Methamphetamine Abusers
Abstract
OBJECTIVE: Methamphetamine has raised concerns because it may be neurotoxic to the human brain. Although prior work has focused primarily on the effects of methamphetamine on dopamine cells, there is evidence that other neuronal types are affected. The authors measured regional brain glucose metabolism, which serves as a marker of brain function, to assess if there is evidence of functional changes in methamphetamine abusers in regions other than those innervated by dopamine cells. METHOD: Fifteen detoxified methamphetamine abusers and 21 comparison subjects underwent positron emission tomography following administration of [18F]fluorodeoxyglucose. RESULTS: Whole brain metabolism in the methamphetamine abusers was 14% higher than that of comparison subjects; the differences were most accentuated in the parietal cortex (20%). After normalization for whole brain metabolism, methamphetamine abusers exhibited significantly lower metabolism in the thalamus (17% difference) and striatum (where the differences were larger for the caudate [12%] than for the putamen [6%]). Statistical parametric mapping analyses corroborated these findings, revealing higher metabolism in the parietal cortex and lower metabolism in the thalamus and striatum of methamphetamine abusers. CONCLUSIONS: The fact that the parietal cortex is a region devoid of any significant dopaminergic innervation suggests that the higher metabolism seen in this region in the methamphetamine abusers is the result of methamphetamine effects in circuits other than those modulated by dopamine. In addition, the lower metabolism in the striatum and thalamus (major outputs of dopamine signals into the cortex) is likely to reflect the functional consequence of methamphetamine in dopaminergic circuits. These results provide evidence that, in humans, methamphetamine abuse results in changes in function of dopamine- and nondopamine-innervated brain regions.
Methamphetamine is a highly addictive drug (1), and methamphetamine abuse has risen substantially in several areas of the United States (2, 3) and the world (4). As methamphetamine abuse rises, concern about its potential neurotoxic effects on human abusers increases, since methamphetamine administration has been shown to produce long-lasting damage to select cell populations in the brains of laboratory animals. Specific damage has been documented in dopamine cells (5, 6). Methamphetamine has consistently been reported to cause persistent decreases in markers of dopamine cell function and structure (7). Moreover, these changes have been associated with long-lasting motor deficits, which indicate that the neurotoxic effects are functionally significant (8). There is also evidence that methamphetamine causes decreases in markers of serotonin cell function (9) and that it also affects nonmonoaminergic cortical neurons (10, 11). Data from human studies are very limited, and both postmortem as well as imaging studies have mainly focused on the effects of methamphetamine on dopamine cells. These studies have shown a marked reduction in dopamine transporters, which serve as markers for dopamine cell terminals in methamphetamine abusers (12–14). However, there are no data on the effects of methamphetamine in nondopamine neurons in humans. The purpose of this study was to assess whether there are changes in brain regions other than those innervated by dopamine.
Following administration of [18F]fluorodeoxyglucose (FDG), positron emission tomography (PET) was used to measure regional brain glucose metabolism. Cerebral glucose metabolism serves as a marker of neuronal activity (15) and is a sensitive indicator of brain dysfunction (16, 17). The methamphetamine abusers and 18 of the comparison subjects also underwent PET imaging to measure dopamine transporter levels in the brain. The results from these measures, which showed marked striatal dopamine transporter reductions in methamphetamine abusers, are reported in a separate article in this issue of the Journal (18).
Method
Participants
Fifteen subjects (six men and nine women; mean age=32 years, SD=7) who fulfilled DSM-IV criteria for methamphetamine dependence were enrolled in the study. Twelve subjects were tested within 2 weeks to 5 months of their last episode of methamphetamine abuse, and the other three were tested between 11 and 35 months after their last episode of methamphetamine abuse. Methamphetamine abusers were included in the study if their average methamphetamine use involved at least 0.5 gram/day, at least 5 days per week, for at least 2 years. Subjects were also required to have abstained from methamphetamine use for at least 2 weeks, which we confirmed by conducting a urine toxicology screening examination. Methamphetamine abusers were excluded from the study if they were seropositive for HIV or had a history of comorbid psychiatric illness or neurological disorder, abnormal results on laboratory screening tests, current or lifetime history of addiction to drugs other than methamphetamine or nicotine, or a history of head trauma with loss of consciousness for more than 30 minutes. Subjects were recruited from several local drug rehabilitation centers in the Los Angeles area. After potential research subjects underwent an initial telephone or an on-site face-to-face evaluation, a physician (L.C.) conducted a detailed medical and drug use history and physical and neuropsychiatric evaluations. Blood test screenings, including HIV serology, were conducted to determine whether abnormalities were present. Diagnosis and exclusion criteria were corroborated by another physician (M.J.S., G.-J.W., or D.F.).
The comparison subjects consisted of 21 healthy volunteers (15 men and six women; mean age=31 years, SD=8) who responded to a local advertisement. Exclusion criteria were the same as those for methamphetamine abusers except the current or lifetime history of addiction to drugs included dependence or abuse of methamphetamine. As was done for the methamphetamine abusers, a physician (G.-J.W. or D.F.) conducted a complete medical, neurological, and psychiatric examination to ensure lack of disease. The comparison subjects also underwent the same laboratory screening tests (except for HIV serology) as those given to the methamphetamine abusers.
No subject was taking medication at the time of the study, and prescan urine tests were conducted to ensure absence of psychoactive drug use in the comparison subjects and methamphetamine abusers. Written informed consent was obtained from the subjects after the procedures had been fully explained. The study was approved by the institutional review boards at Brookhaven National Laboratory, the State University of New York at Stony Brook, and Harbor-UCLA Medical Center.
Neuropsychological Evaluation
Within 2 weeks of the PET scans, the methamphetamine abusers were administered a neuropsychological battery (19) that measured 1) motor function (by means of the Timed Gait and Grooved Pegboard tasks); 2) attention (assessed with the California Computerized Assessment Package [20], Symbol Digit Modalities Test, Trail Making Test, and Stroop Test); 3) memory (through the Rey Auditory Verbal Learning Test); 4) mood (subjects were administered the Center for Epidemiologic Studies Depression Scale [21]); and 5) general intelligence (assessed with the New Adult Reading Test Revised).
Scans
PET scans were performed with a CTI 931 scanner (Siemens, Knoxville, Tenn.) (15 slices, spatial resolution: 6 × 6 × 6.5 mm full width at half maximum). Details on procedures for positioning, arterial and venous catheterization, quantification of radiotracer, and transmission and emission scans have been published (22). Briefly, one 20-minute emission scan was taken 35 minutes after an intravenous injection of 4–6 mCi of FDG. During the study, subjects were kept lying in the PET camera with their eyes open; the room was dimly lit and noise was kept to a minimum. A nurse remained with the subjects throughout the procedure to ensure that the subject did not fall asleep during the study.
Image and Data Analysis
Regions of interest were selected by using a previously published template that locates 114 regions of interest (22). The regions of interest corresponding to the same anatomical regions were averaged to obtain measures for 10 composite brain regions and one for global metabolism (average metabolism in the 15 planes scanned). To minimize the variation effect of whole brain metabolism on the regional measures, we computed the ratio of the regional to the global metabolic measures to obtain relative measures of metabolism.
Differences between groups in absolute and relative metabolic measures were tested with two-tailed Student’s t tests. Pearson product-moment correlation analyses were performed between the regional metabolic measures with between-group differences and 1) neuropsychological test scores, 2) the years and doses of methamphetamine used, and 3) days since last methamphetamine use. To correct for multiple comparisons, we set the significance threshold at p<0.01. We chose this criterion of significance as being intermediate between the p<0.05 considered significant for an individual variable and the p<0.005 value required by the Bonferroni adjustment. The Bonferroni criterion assumes that variables are independent (23), but regional metabolic values are highly dependent on one another (24).
Metabolic images were also analyzed by using the software package for statistical parametric mapping SPM 98 (25). Statistical parametric maps were displayed in coronal, transverse, and sagittal views, with only those pixels that reached a statistical significance of p<0.005 and a contiguous count criterion of at least 250 pixels per cluster showing.
Results
Global metabolic rate was significantly higher (14%) for the methamphetamine abusers (mean=38.4 μmol/100 g/minute, SD=8) than for the comparison subjects (33.6 μmol/100 g/minute, SD=5) (t=2.2, df=34, p<0.05) (Figure 1). Analysis of the regional measures showed that relative to the comparison subjects, metabolism in the parietal cortex was 20% higher in the methamphetamine abusers, which was a significant difference (Figure 2). While not achieving significance, higher metabolism (differences of 11%–12%) was seen in the frontal cortex (t=2.1, df=34, p<0.05), temporal cortex (t=2.0, df=34, p<0.05), occipital cortex (t=2.4, df=34, p<0.05), and hippocampus (t=2.2, df=34, p<0.05) of the methamphetamine abusers. After normalizing for whole brain metabolism (relative metabolism), the only brain region in which the methamphetamine abusers showed significantly higher metabolism was the parietal cortex, where metabolism was 5% higher (Figure 3). However, the analysis of the relative measures also revealed that the methamphetamine abusers had significantly lower metabolism in the thalamus, caudate, and putamen (differences of 17%, 12%, and 6%, respectively). To determine if metabolic changes in the caudate were significantly larger than those in the putamen, we conducted a factorial (comparison subjects versus methamphetamine abusers) repeated measure (caudate versus putamen) ANOVA. This showed a significant diagnosis-by-region interaction effect (F=4.8, df=1, 34, p<0.05), indicating that the differences between comparison subjects and the methamphetamine abusers were greater in the caudate than in the putamen.
To assess if there was any evidence of recovery with detoxification, we conducted a separate analysis that included only the three subjects who had been detoxified for 11 months or longer; similar results were found as those obtained for the whole group. These long-detoxified methamphetamine abusers had significantly lower relative metabolism in the caudate than comparison subjects (12% difference; t=3.6, df=22, p<0.002) and, although not significant, also had global metabolism that was 13% higher and relative metabolism in the thalamus that was 10% lower. We also measured the correlations between the metabolic measures that differed between the groups and days since last methamphetamine use; none were significant.
There were no significant correlations between whole brain metabolism and neuropsychological scores, but relative metabolism in the parietal cortex was significantly correlated with performance on the Grooved Pegboard task (r=0.73, df=14, p<0.003); there were no significant correlations between neuropsychological scores and thalamic or striatal metabolic measures. Whole brain metabolism was negatively correlated with years of methamphetamine use (r=–0.62, df=14, p<0.02), but this effect was not significant after covarying for age. Correlations between metabolic measures and methamphetamine dose were not significant.
To determine if gender differences between groups (the methamphetamine group had a larger proportion of women [60%] than did the comparison group [28%]) affected the results, we conducted separate comparisons for each gender. The findings were consistent with those when both genders were included. The women (nine methamphetamine abusers and six comparison subjects) had higher whole brain metabolism (t=2.2, df=13, p<0.05) and lower relative metabolism in the thalamus (t=3.8, df=13, p<0.002) and caudate (t=3.0, df=13, p<0.01); the men (six methamphetamine abusers and 15 comparison subjects) had lower relative metabolism in the thalamus (t=3.6, df=19, p<0.002), caudate (t=4.4, df=19, p<0.001), and putamen (t=2.2, df=19, p<0.05) (data not shown).
Statistical parametric mapping analyses revealed significant differences between the comparison subjects and the methamphetamine abusers that corroborated the findings from the region of interest analysis. Methamphetamine abusers had significantly higher metabolism than comparison subjects in the parietal cortex (Brodmann’s areas 39 and 40) and significantly lower metabolism in the thalamus, caudate, and putamen (Figure 4).
Discussion
This study documented significantly higher whole brain metabolism that was most marked in the parietal cortex and significantly lower relative metabolism in the thalamus and striatum of detoxified methamphetamine abusers. These metabolic differences were present even in the three methamphetamine abusers who had been detoxified for at least 11 months. These findings are similar to those that have been reported in laboratory animals, which showed persistent reductions in subcortical metabolism after repeated administration of methamphetamine to rats (26). The striatum receives direct projections from dopamine mesencephalic cells, which in animals have been shown to be sensitive to methamphetamine’s neurotoxic effects (5–7); hence, the reduction in brain metabolism could reflect methamphetamine-induced disruption to these cells. The thalamus, on the other hand, mainly receives dopamine signals from the striatum (27). Thus, one could also postulate that the lower metabolism in the thalamus of the methamphetamine abusers reflects disruption in dopamine-linked pathways. Thus, these findings provide evidence that methamphetamine at the doses abused by humans causes long-lasting changes in brain metabolism in both regions that are directly or indirectly connected to dopamine pathways (striatum and thalamus) as well as regions that are not (parietal cortex).
The finding of brain hypermetabolism in the methamphetamine abusers was unexpected, since most studies have revealed decrements in brain metabolism in drug abusers (detoxified alcoholics or cocaine abusers) (28). A review of the literature showed that brain disease states for which higher metabolism has been reported, such as head trauma (29) and radiation damage (30, 31), usually involve inflammation and gliosis (32). Studies in laboratory animals have documented that methamphetamine induces gliosis (33, 34), and although such an effect has not been directly documented in the human brain, studies with magnetic resonance spectroscopy have shown increases in the concentration of myo-inositol, which serves as a glial marker, in methamphetamine abusers (35). Thus, one could speculate that the hypermetabolism in the methamphetamine abusers could reflect gliosis/inflammation, and studies to evaluate this possibility merit further investigation.
The hypermetabolic activity in the brain of the methamphetamine abusers was most prominent in the parietal cortex (association cortex), a brain region that is poorly innervated by dopamine terminals. Hence, the hypermetabolism most likely reflects the effects of methamphetamine on neuronal types or circuits other than dopamine. In laboratory animals, the parietal cortex was found to be particularly sensitive to neurotoxicity from methamphetamine (36). In the parietal cortex, methamphetamine produces degeneration of myelinated processes, damages pyramidal glutamatergic cells (10, 11), and damages serotonergic terminals (37). Methamphetamine also increases glutamate receptors in the parietal cortex (38), which would make it more sensitive to glutamate excitotoxicity. Unfortunately, none of the neuropsychological tests used to evaluate the methamphetamine abusers specifically targeted functions of the parietal cortex. Nonetheless, we found that in the methamphetamine abusers metabolism in the parietal cortex was correlated with performance on the Grooved Pegboard task. Although the Grooved Pegboard is considered a motor task (22), there is a spatial component to it: the subject has to make a decision about where to insert the pegs and then orient the grooves in a certain way in order for them to match and be inserted into the holes. Although preliminary in its interpretation, this significant association suggests that hyperactivity of the parietal cortex may be functionally significant.
Methamphetamine abusers also showed significantly lower relative metabolism in the thalamus and striatum. This pattern is reminiscent of the metabolic abnormalities reported in patients with atypical Parkinson’s disease (who also show relatively lower thalamic and striatal metabolism [39]) but differs from abnormalities reported in patients with Parkinson’s disease (who show either no metabolic changes [40] or higher striatal and thalamic metabolism [41]). The findings of dopamine transporter reductions in methamphetamine abusers (12–14) also more closely resemble the findings from patients with atypical Parkinson’s disease than patients with idiopathic Parkinson’s in that the former, like the methamphetamine abusers, exhibit equivalent dopamine transporter reductions in the caudate and putamen (42), whereas the patients with Parkinson’s disease have greater dopamine transporter reductions in the putamen than in the caudate (43, 44). This is relevant because patients with atypical Parkinson’s disease in addition to dopamine cell damage have degeneration of pallidal efferents, whereas patients with idiopathic Parkinson’s have a predominant involvement of dopamine cells. Moreover, in patients with atypical Parkinson’s disease, the decrements in subcortical metabolism are believed to reflect degeneration of striatal efferents (45). Thus, one could postulate that the abnormalities in the striatum and thalamus in the methamphetamine abusers reflect not only methamphetamine’s effects in dopamine terminals (12–14) but also its effects on striatal efferents. In fact, animal studies have shown that methamphetamine, in addition to damaging dopamine terminals, also induces degeneration of striatal efferents (46). Thus, the results from this study coupled with the findings of dopamine transporter reduction in methamphetamine abusers (12–14) should alert clinicians of the possibility of a higher risk for neurodegenerative diseases in these subjects.
The pattern of regional brain metabolic abnormalities seen in these methamphetamine abusers differs from that reported after acute methamphetamine administration in humans, which showed decreases in cortical metabolism and increases in cerebellar metabolism (47). This indicates that the regions that may be most sensitive to acute administration are not necessarily the same as those that are vulnerable to the long-term effects of methamphetamine.
In generalizing the findings from this study it is relevant to address its limitations. This study was done with outpatients, and thus it is not possible to determine with certainty that the period since last methamphetamine use was accurate. Also, in this type of clinical study there are inaccuracies regarding exact amount and histories of drug use by the substance abusers as well as denial of drug use by the comparison subjects. Although we performed a careful physical examination and obtained routine laboratory tests, we did not test for all potential confounding diseases (e.g., HIV in the comparison subjects). Neurocognitive tests were only performed by the methamphetamine abusers, and thus it is not possible to determine the extent to which the groups differed. There are also confounds from differences in the groups investigated; the percentage of smokers in the methamphetamine group (73%) was higher than in the comparison group (24%), and although we excluded subjects if they were addicted to drugs apart from nicotine (or methamphetamine for the methamphetamine abusers), it is likely that the methamphetamine abusers consumed larger amounts of other drugs (i.e., alcohol, marijuana) than the comparison group.
In summary, the results from this study provide evidence that methamphetamine at doses abused by humans induces long-lasting metabolic changes in brain regions neuroanatomically connected with dopamine pathways but also in areas that are not innervated by dopamine. Prospective studies coupled with neuropsychological assessment of methamphetamine abusers are required to determine if these changes recover and if they predispose to neurodegenerative diseases.
Received April 26, 2000; revision received Sept. 8, 2000; accepted Sept. 13, 2000. From the Brookhaven National Laboratory; the Department of Psychiatry, State University of New York at Stony Brook; and the UCLA Departments of Neurology and Psychiatry. Address reprint requests to Dr. Volkow, Brookhaven National Laboratory, Building 490, P.O. Box 5000, Upton, NY 11973; [email protected] (e-mail). Supported in part by a U.S. Department of Energy contract (ACO2-98-CH-10886), grants from the National Institute on Drug Abuse (DA-7092-01 and DA-00280) and the Office of National Drug Control Policy, and a General Clinical Research Center grant (RR-10710) for University Hospital Stony Brook from NIH. The authors thank David Schlyer and Robert Carciello for cyclotron operations; Donald Warner for PET operations; Colleen Shea, Victor Garza, Robert MacGregor, David Alexoff, and Payton King for radiotracer preparation and analysis; and Pauline Carter, Paula Cervany, Noelwah Netusil, and Naomi Pappas for patient care.
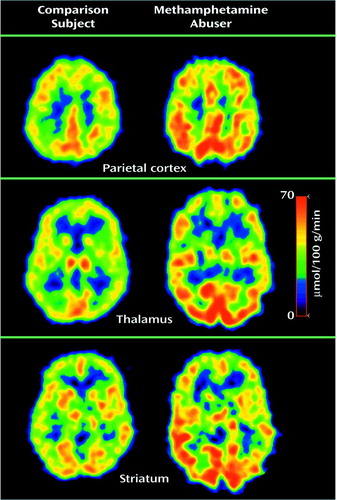
Figure 1. Parietal Cortex, Thalamic, and Striatal Metabolism in a Detoxified Methamphetamine Abuser and in an Age-Matched Comparison Subject With No History of Drug Abusea
aThe upper images were taken at the level of the centrum semiovale, where the parietal cortex can be visualized. Note the higher metabolism in the cortex and the lower metabolism in the thalamus and the caudate in the methamphetamine abuser.
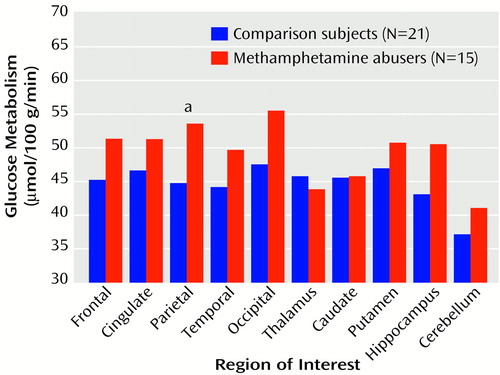
Figure 2. Regional Cerebral Glucose Metabolism in Detoxified Methamphetamine Abusers and Comparison Subjects With No History of Drug Abuse
aSignificant difference between groups (t=2.9, df=34, p<0.01).
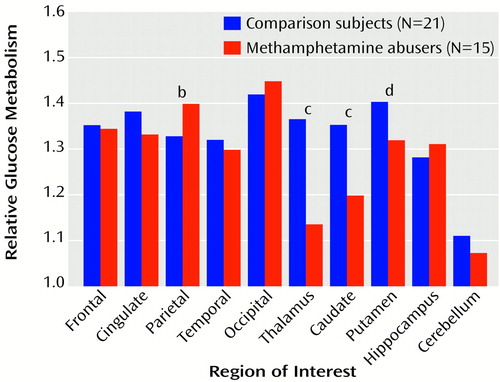
Figure 3. Relative Cerebral Glucose Metabolisma in Detoxified Methamphetamine Abusers and Comparison Subjects With No History of Drug Abuse
aRegional metabolism normalized for global brain activity (region of interest/global).
bSignificant difference between groups (t=3.7, df=34, p<0.001).
cSignificant difference between groups (t=5.5, df=34, p<0.0001).
dSignificant difference between groups (t=2.7, df=34, p<0.01).
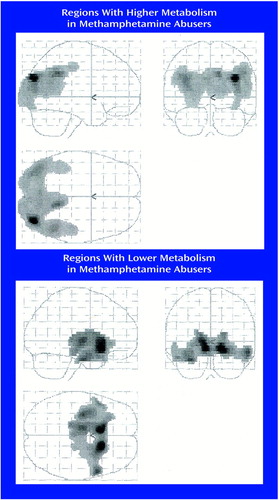
Figure 4. Regions of Significant Differences in Metabolism Between 15 Detoxified Methamphetamine Abusers and 21 Comparison Subjects With No History of Drug Abusea
aStatistical parametric mapping images show areas in which significantly higher and significantly lower metabolism was seen in the methamphetamine abusers relative to the comparison subjects as determined by two-tailed Student’s t tests (df=34). Only pixels that met a significance level of p<0.005 and a contiguous count criterion of at least 250 pixels per cluster are shown.
1. Woolverton WL, Cervo L, Johanson CE: Effects of repeated methamphetamine administration on methamphetamine self-administration in rhesus monkeys. Pharmacol Biochem Behav 1984; 21:737–741Crossref, Medline, Google Scholar
2. Lukas SE: Proceedings of the National Consensus Meeting on the Use, Abuse and Sequelae of Abuse of Methamphetamine With Implications for Prevention, Treatment and Research. DHHS Publication SMA 96–8013. Rockville, Md, Substance Abuse and Mental Health Administration, 1997Google Scholar
3. National Institute on Drug Abuse: Methamphetamine abuse alert. NIDA Notes 1999; 13:15–16Google Scholar
4. Shaw KP: Human methamphetamine-related fatalities in Taiwan during 1991–1996. J Forensic Sci 1999; 44:27–31Medline, Google Scholar
5. Gibb JW, Johnson M, Elayan I, Lim HK, Matsuda L, Hanson GR: Neurotoxicity of amphetamines and their metabolites. NIDA Res Monogr 1997; 173:128–145Medline, Google Scholar
6. Preston KL, Wagner GC, Schuster CR, Seiden LS: Long-term effects of repeated methylamphetamine administration on monoamine neurons in the rhesus monkey brain. Brain Res 1985; 338:243–248Crossref, Medline, Google Scholar
7. Seiden LS, Sabol KE: Methamphetamine and methylenedioxymethamphetamine neurotoxicity: possible mechanisms of cell destruction. NIDA Res Monogr 1996; 163:251–276Medline, Google Scholar
8. Walsh SL, Wagner GC: Motor impairments after methamphetamine-induced neurotoxicity in the rat. J Pharmacol Exp Ther 1992; 263:617–626Medline, Google Scholar
9. Ricaurte GA, Schuster CR, Seiden LS: Long-term effects of repeated methylamphetamine administration on dopamine and serotonin neurons in the rat brain: a regional study. Brain Res 1980; 193:153–163Crossref, Medline, Google Scholar
10. Commins DL, Seiden LS: Alpha-methyltyrosine blocks methylamphetamine-induced degeneration in the rat somatosensory cortex. Brain Res 1986; 365:15–20Crossref, Medline, Google Scholar
11. Ryan LJ, Linder JC, Martone ME, Groves PM: Histological and ultrastructural evidence that d-amphetamine causes degeneration in neostriatum and frontal cortex of rats. Brain Res 1990; 518:67–77Crossref, Medline, Google Scholar
12. Volkow ND, Chang L, Wang G-J Fowler JS, Franceschi D, Gatley SJ, Wong CT, Hitzemann RJ, Pappas NR: In vivo evidence that methamphetamine abuse produces long lasting changes in dopamine transporters in human brain (abstract). J Nucl Med 1999; 40:110Medline, Google Scholar
13. Wilson JM, Kalasinsky KS, Levey AI, Bergeron C, Reiber G, Anthony RM, Schmunk GA, Shannak K, Haycock JW, Kish SJ: Striatal dopamine nerve terminal markers in human, chronic methamphetamine users. Nat Med 1996; 2:699–703Crossref, Medline, Google Scholar
14. McCann UD, Wong DF, Yokoi F, Villemagne V, Dannals RF, Ricaurte GA: Reduced striatal dopamine transporter density in abstinent methamphetamine and methcathinone users: evidence from positron emission tomography studies with [11C]WIN-35,428. J Neurosci 1998; 18:8417–8422Google Scholar
15. Schmidt KC, Lucignani G, Sokoloff L: Fluorine-18-fluorodeoxyglucose PET to determine regional cerebral glucose utilization: a re-examination. J Nucl Med 1996; 37:394–399Medline, Google Scholar
16. Frey KA, Minoshima S, Kuhl DE: Neurochemical imaging of Alzheimer’s disease and other degenerative dementias. Q J Nucl Med 1998; 42:166–178Medline, Google Scholar
17. Kushner M, Reivich M, Fieschi C, Silver F, Chawluk J, Rosen M, Greenberg J, Burke A, Alavi A: Metabolic and clinical correlates of acute ischemic infarction. Neurology 1987; 37:1103–1110Google Scholar
18. Volkow ND, Chang L, Wang G-J, Fowler JS, Leonido-Yee M, Franceschi D, Sedler M, Gatley J, Hitzemann R, Ding Y-S, Logan J, Wong C, Miller EN: Association of lower dopamine transporter levels with psychomotor impairment in methamphetamine abusers. Am J Psychiatry 2001; 158:384–390Google Scholar
19. Lezak MD: Neuropsychological Assessment, 3rd ed. New York, Oxford University Press, 1995Google Scholar
20. Miller EN: California Computerized Assessment Package. Los Angeles, Norland Software, 1990Google Scholar
21. Radloff LS: The CES-D Scale: a self-report depression scale for research in the general population. J Applied Psychol Measurement 1977; 1:385–401Crossref, Google Scholar
22. Wang GJ, Volkow ND, Roque CT, Cestaro VL, Hitzemann RJ, Cantos EL, Levy AV, Dhawan AP: Functional significance of ventricular enlargement and cortical atrophy in normals and alcoholics as assessed by PET, MRI and neuropsychological testing. Radiology 1992; 186:59–65Crossref, Google Scholar
23. Haiz WL: Statistics for the Social Sciences. New York, Holt Reinhart & Winston, 1973Google Scholar
24. Volkow ND, Brodie JD, Wolf AP, Gomez-Mont F, Cancero R, Van Gelder P, Russell JAG, Overall J: Brain organization of schizophrenics. J Cereb Blood Flow Metab 1986; 6:441–446Crossref, Medline, Google Scholar
25. Acton PD, Friston KJ: Statistical parametric mapping in functional neuroimaging: beyond PET and fMRI activation studies. Eur J Nucl Med 1998; 25:663–667Medline, Google Scholar
26. Huang YH, Tsai SJ, Su TW, Sim CB: Effects of repeated high-dose methamphetamine on local cerebral glucose utilization in rats. Neuropsychopharmacology 1999; 21:427–434Crossref, Medline, Google Scholar
27. Scheel-Krüger J: Dopamine-GABA interactions: evidence that GABA transmits, modulates and mediates dopaminergic functions in the basal ganglia and the limbic system. Acta Neurol Scand 1986; 73:9–49Crossref, Google Scholar
28. Gatley SJ, Volkow ND: Addiction and imaging of the living human brain. Drug Alcohol Depend 1998; 51:97–108Crossref, Medline, Google Scholar
29. Kawamata T, Katayama Y, Hovda DA, Yoshino A, Becker DP: Administration of excitatory amino acid antagonists via microdialysis attenuates the increase in glucose utilization seen following concussive brain injury. J Cereb Blood Flow Metab 1992; 12:12–24Crossref, Medline, Google Scholar
30. Sasaki M, Ichiya Y, Kuwabara Y, Yoshida T, Inoue T, Morioka T, Hisada K, Fukui M, Masuda K: Hyperperfusion and hypermetabolism in brain radiation necrosis with epileptic activity. J Nucl Med 1996; 37:1174–1176Google Scholar
31. Fischman AJ, Thornton AF, Frosch MP, Swearinger B, Gonzalez RG, Alpert NM: FDG hypermetabolism associated with inflammatory necrotic changes following radiation of meningioma. J Nucl Med 1997; 38:1027–1029Google Scholar
32. Roh JK, Nam H, Lee MC: A case of central pontine and extrapontine myelinolysis with early hypermetabolism on 18FDG-PET scan. J Korean Med Sci 1998; 13:99–102Crossref, Medline, Google Scholar
33. Escubedo E, Guitart L, Sureda FX, Jimenez A, Pubill D, Pallas M, Camins A, Camarasa J: Microgliosis and down-regulation of adenosine transporter induced by methamphetamine in rats. Brain Res 1998; 814:120–126Crossref, Medline, Google Scholar
34. Sheng P, Cerruti C, Cadet JL: Methamphetamine (METH) causes reactive gliosis in vitro: attenuation by the ADP-ribosylation (ADPR) inhibitor, benzamide. Life Sci 1994; 55:PL51–PL54Google Scholar
35. Ernst T, Chang L, Leonido-Yee M, Speck O: Evidence for long-term neurotoxicity associated with methamphetamine abuse: a 1H MRS study. Neurology 2000; 54:1344–1349Google Scholar
36. Pu C, Broening HW, Vorhees CV: Effect of methamphetamine on glutamate-positive neurons in the adult and developing rat somatosensory cortex. Synapse 1996; 23:328–334Crossref, Medline, Google Scholar
37. Brunswick DJ, Benmansour S, Tejani-Butt SM, Hauptmann M: Effects of high-dose methamphetamine on monoamine uptake sites in rat brain measured by quantitative autoradiography. Synapse 1992; 11:287–293Crossref, Medline, Google Scholar
38. Eisch AJ, O’Dell SJ, Marshall JF: Striatal and cortical NMDA receptors are altered by a neurotoxic regimen of methamphetamine. Synapse 1996; 22:217–225Crossref, Medline, Google Scholar
39. Antonini A, Kazumata K, Feigin A, Mandel F, Dhawan V, Margouleff C, Eidelberg D: Differential diagnosis of parkinsonism with [18F]fluorodeoxyglucose and PET. Mov Disord 1998; 13:268–274Crossref, Medline, Google Scholar
40. Brooks DJ: The early diagnosis of Parkinson’s disease. Ann Neurol 1998; 44(3 suppl 1):S10–S18Google Scholar
41. Eidelberg D, Moeller JR, Dhawan V, Spetsieris P, Takikawa S, Ishikawa T, Chaly T, Robeson W, Margouleff D, Przedborski S: The metabolic topography of parkinsonism. J Cereb Blood Flow Metab 1994; 14:783–801Crossref, Medline, Google Scholar
42. Messa C, Volonte MA, Fazio F, Zito F, Carpinelli A, d’Amico A, Rizzo G, Moresco RM, Paulesu E, Franceschi M, Lucignani G: Differential distribution of striatal [123I]beta-CIT in Parkinson’s disease and progressive supranuclear palsy, evaluated with single-photon emission tomography. Eur J Nucl Med 1998; 25:1270–1276Google Scholar
43. Miller GW, Staley JK, Heilman CJ, Perez JT, Mash DC, Rye DB, Levey AI: Immunochemical analysis of dopamine transporter protein in Parkinson’s disease. Ann Neurol 1997; 41:530–539Crossref, Medline, Google Scholar
44. Rinne JO, Ruottinen H, Bergman J, Haaparanta M, Sonninen P, Solin O: Usefulness of a dopamine transporter PET ligand [(18)F]beta-CFT in assessing disability in Parkinson’s disease. J Neurol Neurosurg Psychiatry 1999; 67:737–741Crossref, Medline, Google Scholar
45. Brooks DJ, Ibanez V, Sawle GV, Playford ED, Quinn N, Mathias CJ, Lees AJ, Marsden CD, Bannister R, Frackowiak RS: Striatal D2 receptor status in patients with Parkinson’s disease, striatonigral degeneration, and progressive supranuclear palsy, measured with 11C-raclopride and positron emission tomography. Ann Neurol 1992; 31:184–192Crossref, Medline, Google Scholar
46. Burrows KB, Meshul CK: High-dose methamphetamine treatment alters presynaptic GABA and glutamate immunoreactivity. Neuroscience 1999; 90:833–850Crossref, Medline, Google Scholar
47. Gouzoulis-Mayfrank E, Schreckenberger M, Sabri O, Arning C, Thelen B, Spitzer M, Kovar KA, Hermle L, Bull U, Sass H: Neurometabolic effects of psilocybin, 3,4-methylenedioxyethylamphetamine (MDE) and d-methamphetamine in healthy volunteers: a double-blind, placebo-controlled PET study with [18F]FDG. Neuropsychopharmacology 1999; 20:565–581Crossref, Medline, Google Scholar