Role of Neuronal VEGF Signaling in the Prefrontal Cortex in the Rapid Antidepressant Effects of Ketamine
Abstract
Objective:
The N-methyl-d-aspartate receptor antagonist ketamine produces rapid and sustained antidepressant actions even in patients with treatment-resistant depression. Vascular endothelial growth factor (VEGF) has been implicated in the effects of conventional monoamine-based antidepressants, but the role of VEGF in the rapid antidepressant actions of ketamine remains unclear. The authors examined whether neuronal VEGF signaling in the medial prefrontal cortex (mPFC) mediates the rapid antidepressant actions of ketamine.
Methods:
The authors used a combination of approaches, including conditional, neuron-specific knockout of VEGF or its receptor, Flk-1; antibody neutralization; viral-mediated knockdown of Flk-1; and pharmacological inhibitors. Further in vitro and in vivo experiments were performed to examine whether neuronal VEGF signaling was required for the neurotrophic and synaptogenic actions of ketamine that underlie its behavioral actions.
Results:
The behavioral actions of systemic ketamine are blocked by forebrain excitatory neuron-specific deletion of either VEGF or Flk-1 or by intra-mPFC infusion of a VEGF neutralizing antibody. Moreover, intra-mPFC infusions of VEGF are sufficient to produce rapid ketamine-like behavioral actions, and these effects are blocked by neuron-specific Flk-1 deletion. The results also show that local knockdown of Flk-1 in mPFC excitatory neurons in adulthood blocks the behavioral effects of systemic ketamine. Moreover, inhibition of neuronal VEGF signaling blocks the neurotrophic and synaptogenic effects of ketamine.
Conclusions:
Together, these findings indicate that neuronal VEGF–Flk-1 signaling in the mPFC plays an essential role in the antidepressant actions of ketamine.
Major depressive disorder is one of the most widespread psychiatric illnesses, affecting an estimated 300 million people worldwide (1) and carrying enormous personal and socioeconomic consequences (2). Neuroimaging studies demonstrate reduced volume of the prefrontal cortex (PFC) and hippocampus (3, 4), where neuronal atrophy and glial loss have been reported in postmortem brains from depressed subjects and rodent chronic stress models (5, 6). Although the mechanisms underlying the pathophysiology and treatment of depression are still unknown, there is mounting evidence indicating a role of growth factors, notably brain-derived neurotrophic factor (BDNF) and vascular endothelial growth factor (VEGF). Chronic stress and depression decrease the expression and function of BDNF and/or VEGF in the PFC and hippocampus (7–11); VEGF is also decreased in the CSF of patients who have attempted suicide (12). In contrast, chronic, but not acute, treatment with typical antidepressants, notably monoamine reuptake inhibitors, increases BDNF and VEGF expression (7, 13–17), and blockade of BDNF or VEGF signaling attenuates the antidepressant effects of these treatments (13, 17, 18). These findings support a neurotrophic hypothesis of depression that reduced neurotrophic factor levels are strongly linked with the structural alterations caused by chronic stress and depression and, conversely, that antidepressants act at least in part by producing the opposite effects via induction of BDNF and VEGF expression.
In contrast to the delayed response of weeks to months for the action of typical antidepressants, a single subanesthetic dose of ketamine, an N-methyl-d-aspartate receptor antagonist, produces rapid (within hours) and sustained (up to 1 week) antidepressant actions even in patients with treatment-resistant depression (5, 19). Preclinical studies have reported that low-dose ketamine produces rapid, sustained antidepressant behavioral responses and increases the number and function of synapses in medial PFC (mPFC) pyramidal neurons, reversing the synaptic loss caused by chronic stress (20, 21); these effects are dependent on the processing and activity-dependent release of BDNF (22, 23). Together, these findings indicate that the neurotrophic responses are associated with the behavioral actions of ketamine, but it remains unclear whether VEGF signaling is also involved. VEGF is a pleiotropic growth factor expressed by neurons and glia, as well as vascular endothelial cells (18), and has potent neurotrophic activity (24). Here, we examined the role of neuronal VEGF signaling in the behavioral and neurotrophic effects of ketamine.
Methods
Animals
Male wild-type CaMKIIα-Cre (25), Flk-1flox/flox (26), VEGFflox/flox (27), CaMKIIα-Cre:Flk-1flox/flox (hereafter, Flk-1neuron−/−), and CaMKIIα-Cre:VEGFflox/flox (hereafter, VEGFneuron−/−) mice and Sprague-Dawley rats were used. Animal use and procedures were in accordance with National Institutes of Health guidelines and were approved by the Yale University Animal Care and Use Committee.
Surgery and Drug Infusion
Guide cannulas were implanted bilaterally into the mPFC (rats, 3.0 mm rostral, ±1.0 mm lateral, 4.0 mm ventral to the bregma; mice, 1.8 mm rostral, ±0.4 mm lateral, 2.5 mm ventral to the bregma) or dorsal striatum (rats, 1.6 mm rostral, ±1.9 mm lateral, 5.0 mm ventral to the bregma). Rats were bilaterally infused with a VEGF neutralizing antibody or rat recombinant VEGF164 using an injector that protruded 0.5 mm beyond the tip of the guide cannula (0.5 μL/side; 0.25 μL/min). Mice were bilaterally infused with ketamine or mouse recombinant VEGF164 using an injector that protruded 0.3 mm beyond the tip of the guide cannula (0.2 μL/side; 0.2 μL/min).
AAV2shFlk-1 Preparation and Intra-mPFC Viral Infusion
Sense and antisense oligonucleotides encoding the Flk-1 short hairpin RNA (shFlk-1) were ligated into a plasmid (pshFlk-1) designed to restrict shRNA expression to cells that express Cre recombinase, as has been previously reported (28). This plasmid allows U6 promoter-dependent shFlk-1 expression only when cytomegalovirus promoter-enhanced green fluorescent protein (CMV-EGFP) reporter/stop cassette is eliminated by Cre-dependent recombination.
shFlk-1:
Sense: 5′-TTAAACCGGGATGTGAAACCCTTTCTTCAAGAGAGAAAGGGTTTCACATCCCGGTTTATTTTTTC-3′
Antisense: 5′-TCGAGAAAAAATAAACCGGGATGTGAAACCCTTTCTCTCTTGAAGAAAGGGTTTCACATCCCGGTTTAA-3′
To package into AAV2shFlk-1, HEK293 cells were transfected with pHelper, pAAV2-RC, and pshFlk-1 constructs using Lipofectamine 2000 (Life Technologies, Carlsbad, Calif.). Forty-eight hours after transfection, AAV2shFlk-1 particles were purified, resuspended, and stored at −80°C. Mice were infused with AAV2shFlk-1 bilaterally into the mPFC (2.0 mm rostral, ±0.3 mm lateral, 2.8 mm ventral to the bregma; 1 μL; 0.15 μL/min).
Behavioral Testing
A forced swim test, novelty-suppressed feeding test, and female urine sniffing test were performed, and these have been described elsewhere (17, 22, 28–31). In the forced swim test, 24 hours after a preswim, each animal was placed in a swim cylinder for 10 minutes and videotaped. The immobility time was scored during minutes 2 through 6. In the novelty-suppressed feeding test, animals were food-deprived overnight and placed in an open field with a small amount of food in the center. The latency to feed was measured. In the female urine sniffing test, animals were exposed to a cotton-tipped applicator infused with fresh urine from females of the same strain for 5 minutes. The time spent sniffing the cotton-tipped applicator was measured. In the tail suspension test, each mouse was suspended by its tail for 6 minutes and videotaped. The immobility time was measured during the last 4 minutes. In the locomotor activity test, each animal was placed in a testing chamber for 20 minutes. The total distance traveled (mice) or the number of beam breaks (rats) was measured.
Immunohistochemistry
After 3,3′-diaminobenzidine staining, the number of c-Fos-positive cells was counted in the regions near the infusion sites in the mPFC from two coronal sections (40 μm) in each rat.
Immunofluorescence
Free-floating sections (30 μm) were incubated with the following primary antibodies: rabbit anti-CaMKII, mouse anti-CaMKII, goat antimouse Flk-1, mouse antiglucose transporter 1, and rabbit anti-VEGF. They were then incubated with secondary antibodies. To quantify knockdown, the number of Flk-1-positive cells were counted and calculated as the percentage of total DsRed+ cells.
Golgi Staining and Spine Density Analysis
Golgi staining was performed using the FD Rapid GolgiStain kit (FD NeuroTechnologies, Columbia, Md.) according to the manufacturer’s instructions. Spine density on the primary and secondary dendritic branches of the apical tuft of layer V pyramidal neurons in the infralimbic and prelimbic regions of the mPFC was analyzed using Neurolucida 10 (MBF Bioscience, Williston, Vt.).
Sholl Analysis
Dendritic complexity in primary cortical neurons was analyzed as described elsewhere (32). Cortical neurons were dissected from E18 rat embryos, incubated with AAV2-EGFP for 72 hours, and treated with 500 nM of ketamine or 50 ng/mL of VEGF with or without the Flk-1 inhibitor ZM323881. After 24 hours of incubation, the number of dendritic crossings 50 μm and 100 μm from the soma was measured.
Statistics
Data are expressed as means and standard deviations. Data were analyzed by unpaired t test, one-way analysis of variance (ANOVA), or two-way ANOVA followed by Fisher’s least significant difference test using GraphPad Prism 6 (GraphPad Software). Differences with p<0.05 were considered statistically significant.
Detailed methods are available in the online supplement.
Results
Neuronal VEGF Signaling and Antidepressant-Like Behavioral Effects of Ketamine
To investigate the role of neuronal VEGF signaling in the antidepressant-like behavioral effects of ketamine, we engineered mice with neuron-specific deletion of the VEGF and Flk-1 in the forebrain, referred to as Flk-1neuron−/− and VEGFneuron−/− (Figure 1A and 1B). Both Flk-1 and VEGF are expressed in pyramidal neurons in the mPFC and hippocampus, and neuronal deletion of Flk-1 and VEGF in the Flk-1neuron−/− and VEGFneuron−/− mice, respectively, was confirmed by immunohistochemistry (see Figures S1 through S4 in the online supplement). Flk-1neuron−/− and VEGFneuron−/− mice exhibited normal ambulation and mild anxiety-like behaviors (see Figure S5 in the online supplement). Flk-1neuron−/−, VEGFneuron−/−, and littermate controls (Flk-1flox/flox and VEGFflox/flox, but CaMKIIα-Cre negative) were injected intraperitoneally with ketamine (10 mg/kg) and tested in the forced swim test and novelty-suppressed feeding test 2 and 5 days later, respectively (Figure 1C). In the forced swim test, a model of behavioral despair, a single dose of ketamine significantly decreased immobility in control mice, and this effect was fully blocked in Flk-1neuron−/− or VEGFneuron−/− mice (Figure 1D and 1G); similar effects were observed in the preswim test conducted 1 day after ketamine infusion (see Figure S6A and S6B in the online supplement). There were no significant differences in immobility between saline-treated Flk-1flox/flox and Flk-1neuron−/− mice or between saline-treated VEGFflox/flox and VEGFneuron−/− mice. In the novelty-suppressed feeding test, a model in which increased latency to feed is associated with anxiety, a single dose of ketamine decreased latency to feed in Flk-1flox/flox control mice; Flk-1neuron−/− mice, both saline and ketamine injected, displayed significantly decreased latency compared with saline-injected Flk-1flox/flox control mice (Figure 1E), making the results difficult to interpret. No effect was observed in saline-injected VEGFneuron−/− mice in latency to feed, and the ketamine-mediated reduction in latency to feed was completely blocked (Figure 1H). There were no significant effects of ketamine or Flk-1neuron−/− and VEGFneuron−/− deletion on home cage feeding, demonstrating that these treatments do not produce a general effect on feeding behavior (Figure 1F and 1I).
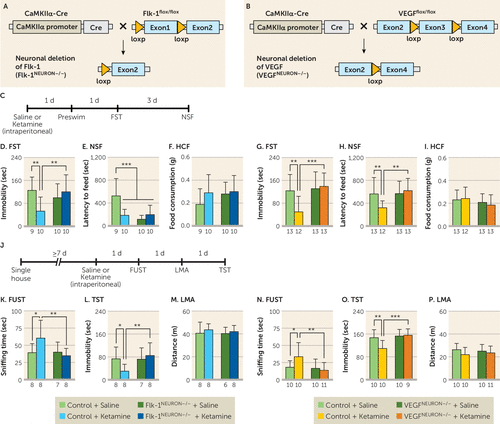
FIGURE 1. Effects of neuron-specific knockout of vascular endothelial growth factor (VEGF) or Flk-1 on the behavioral effects of ketaminea
a Schematic representation of neuronal deletion of Flk-1 (panel A) and VEGF (panel B). Panel C shows the experimental timeline for behavioral testing starting 1 day after intraperitoneal injection of either saline or ketamine (10 mg/kg) in Flk-1neuron−/− mice, VEGFneuron−/− mice, and littermate controls. Panels D and G show immobility time in the forced swim test (FST) 2 days after intraperitoneal injection (panel D interaction, F=8.01, df=1, 35, p=0.0077, N=9–10; panel G interaction, F=7.15, df=1, 47, p=0.010, N=12–13). Panels E and H show latency to feed in the novelty-suppressed feeding test (NSF) 5 days after intraperitoneal injection (panel E interaction, F=13.4, df=1, 35, p=0.0008, N=9–10; panel H interaction, F=5.69, df=1, 47, p=0.021, N=12–13). Panels F and I show home cage feeding (HCF) just after the NSF (panel F interaction, F=0.857, df=1, 35, p=0.36, N=9–10; panel I interaction, F=0.476, df=1, 47, p=0.49, N=12–13). Panel J shows the experimental timeline for behavioral testing starting 1 day after intraperitoneal injection of either saline or ketamine (10 mg/kg) in single-housed Flk-1neuron−/− mice, VEGFneuron−/− mice, and littermate controls. Panels K and N show time spent sniffing female urine in the female urine sniffing test (FUST) 1 day after intraperitoneal injection of either saline or ketamine (panel K interaction, F=4.55, df=1, 27, p=0.042, N=7–8; panel N interaction, F=4.15, df=1, 37, p=0.049, N=10–11). Panels L and O show immobility time in the tail suspension test (TST) 3 days after intraperitoneal injection (panel L interaction, F=4.12, df=1, 27, p=0.052, N=7–8; panel O interaction, F=5.96, df=1, 35, p=0.020, N=9–10). Panels M and P show locomotor activity (LMA) 2 days after intraperitoneal injection (panel M, F=0.0696, df=1, 26, p=0.79, N=6–8; panel P, F=0.57, df=1, 37, p=0.45, N=10–11). Data are expressed as means and standard deviations. d=day; sec=second.
*p<0.05. **p<0.01. ***p<0.001.
We also conducted additional tests for reward seeking or anhedonia (female urine sniffing test) and despair-like behavior (tail suspension test), as well as locomotor activity in separate Flk-1neuron−/− and VEGFneuron−/− cohorts (Figure 1J). In the female urine sniffing test, ketamine significantly increased female urine sniffing time in Flk-1flox/flox and VEGFflox/flox control mice but had no effect in Flk-1neuron−/− or VEGFneuron−/− mice (Figure 1K and 1N). In the tail suspension test, ketamine decreased immobility in control mice, and this effect was blocked in Flk-1neuron−/− and VEGFneuron−/− mice (Figure 1L and 1O). There were no effects of genotype or ketamine on locomotor activity (Figure 1M and 1P). These results indicate that neuronal VEGF–Flk-1 signaling in the forebrain contributes to the behavioral actions of ketamine.
Because BDNF signaling is required for the behavioral and synaptic actions of ketamine (22, 23), we also examined the influence of Flk-1 or VEGF deletion on BDNF, total tropomyosin receptor kinase B (TrkB), and phosphorylated/activated TrkB (pTrkB). Immunoblot analysis shows that there were no significant differences in basal levels of BDNF, TrkB, and pTrkB in the PFC of Flk-1neuron−/− or VEGFneuron−/− mice compared with control mice (see Figure S7B through S7K in the online supplement). In addition, there were no significant effects of ketamine (after 1 hour) on levels of BDNF, TrkB, and pTrkB in either Flk-1neuron−/− or VEGFneuron−/− deletion mutant mice or in controls (see Figure S7B through S7K in the online supplement).
Prefrontal VEGF Release and Antidepressant-Like Behavioral Effects of Ketamine
Our previous studies revealed that the mPFC is necessary and sufficient for the actions of ketamine (20, 22, 30). To examine VEGF in the mPFC, rats were infused with a VEGF neutralizing antibody (0.2 μg/side) into the mPFC 30 minutes before intraperitoneal ketamine infusion (10 mg/kg) and were tested 1 and 5 days later (Figure 2A). In control IgG-infused rats, ketamine produced significant behavioral effects in the forced swim test and novelty-suppressed feeding test (Figure 2B and 2C), and these effects were blocked in VEGF neutralizing antibody-infused rats. There were no effects of ketamine and VEGF neutralizing antibody infusion on home cage feeding (Figure 2D). A subset of rats received a second intra-mPFC infusion of the VEGF neutralizing antibody and intraperitoneal injection after a ≥6-day interval and were tested in the female urine sniffing test 1 day later. Ketamine significantly increased time spent sniffing female urine in IgG-infused rats, and VEGF neutralizing antibody completely blocked this effect (Figure 2E). These treatments did not influence locomotor activity conducted 1.5 hours after the female urine sniffing test (Figure 2F). We also show that ketamine significantly induced c-Fos expression, an immediate early gene marker of neuronal activation, in the mPFC of IgG-infused rats, replicating our previous report (30), and VEGF neutralizing antibody infusion blocked this effect (Figure 2I and 2J).
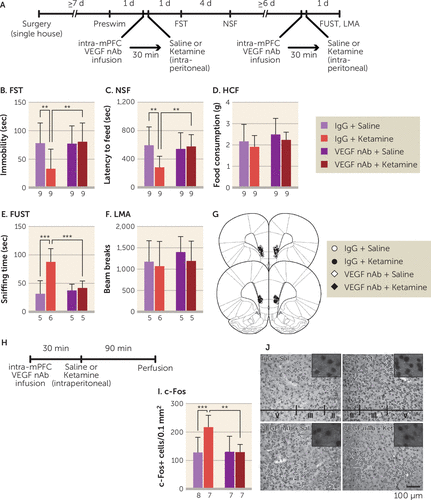
FIGURE 2. Effect of intra–medial prefrontal cortex (mPFC) infusion of vascular endothelial growth factor (VEGF) neutralizing antibody (nAb) on the behavioral effects of ketamine in ratsa
a Panel A shows the experimental timeline for behavioral testing starting 1 day after intra-mPFC infusion of either control IgG (0.2 μg/side) or VEGF nAb (0.2 μg/side) and intraperitoneal injection of either saline or ketamine (10 mg/kg). Panel B shows immobility time in the forced swim test (FST) 1 day after intra-mPFC infusion and intraperitoneal injection (interaction, F=4.64, df=1, 32, p=0.039, N=9). Panel C shows latency to feed in the novelty-suppressed feeding test (NSF) 5 days after intra-mPFC infusion and intraperitoneal injection (interaction, F=6.28, df=1, 32, p=0.018, N=9). Panel D shows home cage feeding (HCF) just after the NSF (interaction, F=0.000248, df=1, 32, p=0.99, N=9). Panel E shows time spent sniffing female urine in the female urine sniffing test (FUST) 1 day after the second intra-mPFC infusion and intraperitoneal injection (interaction, F=10.1, df=1, 17, p=0.0056, N=5–6). Panel F shows locomotor activity (LMA) 1 day after the second intra-mPFC infusion and intraperitoneal injection (interaction, F=0.0583, df=1, 17, p=0.81, N=5–6). Panel G shows the schematic representation of mPFC infusion sites. Panel H shows the experimental timeline for c-Fos quantification. Panel I shows the number of c-Fos-positive cells in the mPFC (interaction, F=7.08, df=1, 25, p=0.013, N=7–8). Panel J shows representative images of c-Fos expression in the mPFC of each group. Data are expressed as means and standard deviations. d=day; min=minute; sec=second.
**p<0.01. ***p<0.001.
Previous studies have demonstrated that signaling required for the synaptic and behavioral actions of ketamine occurs within the first 2 hours (20). Intra-mPFC infusion of VEGF neutralizing antibody 2 hours after ketamine infusion failed to block the behavioral effects of ketamine in the forced swim test, novelty-suppressed feeding test, and female urine sniffing test, indicating that VEGF is required for this initial critical signaling period (see Figure S8 in the online supplement).
To determine whether increased VEGF signaling in the mPFC is sufficient to produce rapid behavioral effects similar to ketamine in the forced swim test, novelty-suppressed feeding test, and female urine sniffing test, rats were infused with recombinant VEGF164, the predominant VEGF isoform, and subjected to behavioral testing (Figure 3A). Intra-mPFC infusion of VEGF164 (5 or 25 ng/side) significantly decreased immobility in the forced swim test (Figure 3B) and latency to feed in the novelty-suppressed feeding test (Figure 3D); there were no effects on locomotor activity or home cage feeding (Figure 3C and 3E). In the female urine sniffing test, a second intra-mPFC VEGF164 infusion (Figure 3A) significantly increased female urine sniffing time (Figure 3F). Intra-mPFC VEGF164 infusion also significantly increased c-Fos-positive cells in the mPFC (Figure 3H through 3J). Moreover, further analysis shows that there were no significant effects of ketamine on VEGF levels in the PFC of C57BL/6J mice 0.5 and 1 hour after injection (see Figure S9 in the online supplement). Together, these findings indicate that elevated VEGF release, but not expression, in the mPFC is necessary and sufficient to produce ketamine-like behavioral actions via increased neuronal activation.
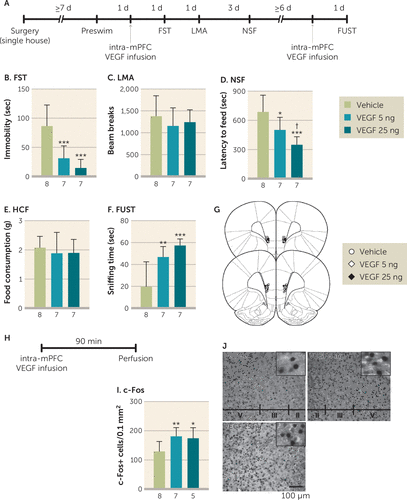
FIGURE 3. Behavioral actions of intra–medial prefrontal cortex (mPFC) infusion of vascular endothelial growth factor (VEGF)164 in ratsa
a Panel A shows the timeline for behavioral testing starting 1 day after intra-mPFC infusion of either vehicle or VEGF164 (5 or 25 ng/side). Panel B shows immobility time in the forced swim test (FST) 1 day after intra-mPFC infusion (F=15.6, df=2, 19, p<0.0001, N=7–8). Panel C shows locomotor activity (LMA) 2 days after intra-mPFC infusion (F=0.598, df=2, 19, p=0.56, N=7–8). Panel D shows latency to feed in the novelty-suppressed feeding test (NSF) 5 days after intra-mPFC infusion (F=11.7, df=2, 19, p=0.0005, N=7–8). Panel E shows home cage feeding (HCF) just after the NSF (F=0.298, df=2, 19, p=0.75, N=7–8). Panel F shows time spent sniffing female urine in the female urine sniffing test (FUST) 1 day after the second intra-mPFC infusion (F=12.5, df=2, 19, p=0.0003, N=7–8). Panel G shows the schematic representation of mPFC infusion sites. Panel H shows the timeline for c-Fos quantification. Panel I shows the number of c-Fos-positive cells in the mPFC (F=5.18, df=2, 17, p=0.018, N=5–8). Panel J shows the representative images of c-Fos expression in the mPFC of each group. Data are expressed as means and standard deviations. d=day; min=minute; sec=second.
*p<0.05. **p<0.01. ***p<0.001. †p<0.05 relative to 5 ng of VEGF164.
To investigate regional selectivity, we tested infusions of VEGF164 (25 ng/side) into the dorsal striatum on the same behaviors (see Figure S10 in the online supplement). Intrastriatal infusion of VEGF164 did not produce any significant behavioral changes.
Neuronal Flk-1 Signaling in the mPFC and the Antidepressant-Like Behavioral Effects of Ketamine
First, we examined the effects of intra-mPFC ketamine infusion (10 ng/side) in Flk-1neuron−/− mice (see Figure S11A in the online supplement). In the forced swim test, intra-mPFC ketamine infusion significantly decreased immobility in control mice but had no effects in Flk-1neuron−/− mice (see Figure S11B in the online supplement); similar effects were observed in the preswim test (see Figure S6C in the online supplement). Intra-mPFC ketamine infusion did not influence locomotor activity (see Figure S11C in the online supplement). In the female urine sniffing test, intra-mPFC ketamine infusion significantly increased time spent sniffing female urine in control mice, and this effect was blocked in Flk-1neuron−/− mice (see Figure S11D in the online supplement). We also found that the behavioral effects of intra-mPFC VEGF164 infusion (5 ng/side) in the forced swim test and female urine sniffing test were blocked in Flk-1neuron−/− mice (see Figure S6D and Figure S11F through S11I in the online supplement).
To confirm that prefrontal VEGF–Flk-1 signaling specifically in mPFC excitatory neurons mediates the behavioral effects of ketamine in the forced swim test, female urine sniffing test, and novelty-suppressed feeding test, we evaluated the influence of selective Flk-1 knockdown in mPFC CaMKIIα-positive neurons (Figure 4A). The AAV2shFlk-1 construct constitutively expresses DsRed and contains a CMV-EGFP cassette disrupting the expression of shFlk-1, which silences Flk-1 mRNA. Upon Cre-dependent recombination, the CMV-EGFP cassette is excised, allowing expression of DsRed only, along with shFlk-1 (Figure 4B). Infusion of AAV2shFlk-1 into the mPFC of CaMKIIα-Cre (CaMKIIα-CremPFC/AAV2shFlk-1) mice resulted in recombination in the majority of CaMKIIα-positive neurons (EGFP−/DsRed+) in the infralimbic and prelimbic regions of the mPFC, with no recombination observed in the mPFC of AAV2shFlk-1-infused wild-type (WTmPFC/AAV2shFlk-1) mice (EGFP+/DsRed+; Figure 4C). Flk-1 immunostaining demonstrated that the number of Flk-1+/EGFP+/DsRed+ cells in CaMKIIα-CremPFC/AAV2shFlk-1 mice, compared with WTmPFC/AAV2shFlk-1 mice, was decreased by approximately 75% (Figure 4D and 4E). Moreover, the proportions of Flk-1+/EGFP−/DsRed+ cells in either CaMKIIα-CremPFC/AAV2shFlk-1 or WTmPFC/AAV2shFlk-1 mice were less than 10% (Figure 4D). These data confirm that Flk-1 knockdown successfully occurred in most recombined (EGFP−/DsRed+) cells in CaMKIIα-CremPFC/AAV2shFlk-1 mice.
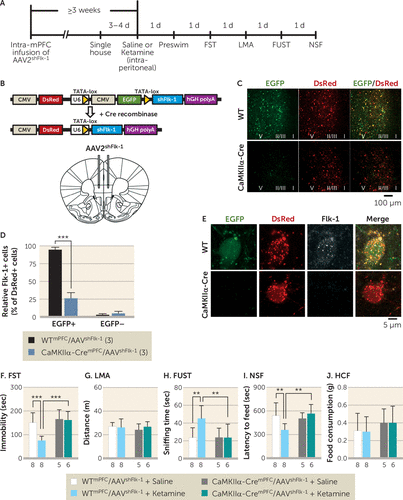
FIGURE 4. Effect of local knockdown of Flk-1 in pyramidal neurons in the medial prefrontal cortex (mPFC) on the behavioral effects of ketaminea
a Panel A shows the experimental timeline for behavioral testing starting ≥3 weeks after intra-mPFC infusion of AAV2shFlk-1 and 1 day after intraperitoneal injection of either saline or ketamine (10 mg/kg). Panel B shows the diagram of plasmid Flk-1 construct and Cre-induced recombination enabling U6-driven expression of Flk-1 short hairpin RNA (shFlk-1). AAV2shFlk-1 was bilaterally infused into the mPFC of wild-type (WT) or CaMKIIα-Cre mice. Panel C shows representative images of the mPFC of WTmPFC/AAV2shFlk-1 (N=16) and CaMKIIα-CremPFC/AAV2shFlk-1 mice (N=11). Approximate laminar regions (I–V) are noted. Panel D shows the relative number of Flk-1+/EGFP+/DsRed+ (t4=13.7, p=0.0002) and Flk-1+/EGFP–/DsRed+ cells (t4=1.06, p=0.348) in the mPFC of WTmPFC/AAV2shFlk-1 (N=3; a mean of 54.7 [SD=22.3] DsRed+ cells per mouse [164 total cells] were analyzed) and CaMKIIα-CremPFC/AAV2shFlk-1 mice (N=3; a mean of 62.3 [SD=12.1] DsRed+ cells per mouse [187 total cells] were analyzed). Panel E shows the representative images of EGFP, DsRed expression, and Flk-1 immunolabeling in mPFC neurons from WTmPFC/AAV2shFlk-1 and CaMKIIα-CremPFC/AAV2shFlk-1 mice. Panel F shows immobility time in the forced swim test (FST) 2 days after intraperitoneal injection (interaction, F=6.88, df=1, 23, p=0.015, N=5–8). Panel G shows locomotor activity (LMA) 3 days after intraperitoneal injection (interaction, F=0.776, df=1, 23, p=0.39, N=5–8). Panel H shows time spent sniffing female urine in the female urine sniffing test (FUST) 4 days after intraperitoneal injection (interaction, F=4.51, df=1, 23, p=0.045, N=5–8). Panel I shows latency to feed in the novelty-suppressed feeding test (NSF) 5 days after intraperitoneal injection (interaction, F=6.84, df=1, 23, p=0.016, N=5–8). Panel J shows home cage feeding (HCF) just after the NSF (interaction, F=0.00778, df=1, 23, p=0.93, N=5–8). Data are expressed as means and standard deviations. CMV=cytomegalovirus; d=day; EGFP=enhanced green fluorescent protein; sec=second.
**p<0.01. ***p<0.001.
In the forced swim test, ketamine significantly decreased immobility in WTmPFC/AAV2shFlk-1 mice, but this effect was blocked in CaMKIIα-CremPFC/AAV2shFlk-1 mice (Figure 4F); similar effects were observed in the preswim test (see Figure S6E in the online supplement); there were no effects on locomotor activity (Figure 4G). In the female urine sniffing test, the time spent sniffing urine that was induced by ketamine in WTmPFC/AAV2shFlk-1 mice was completely blocked in CaMKIIα-CremPFC/AAV2shFlk-1 mice (Figure 4H). In the novelty-suppressed feeding test, ketamine significantly decreased latency to feed in WTmPFC/AAV2shFlk-1 mice, and this effect was fully blocked in CaMKIIα-CremPFC/AAV2shFlk-1 mice (Figure 4I), with no change in home cage feeding (Figure 4J). Unlike the novelty-suppressed feeding results in Flk-1neuron−/− mice (Figure 1C), there was no effect on latency after Flk-1 knockdown in vehicle-treated CaMKIIα-CremPFC/AAV2shFlk-1 mice. These results demonstrate that the behavioral effects of ketamine in these models are mediated by neuronal Flk-1 signaling in the mPFC.
Ketamine’s Neurotrophic and Synaptogenic Effects Require Neuronal VEGF Signaling
Ketamine increases spine density and synaptic function in layer V pyramidal neurons in the mPFC, and this underlies its antidepressant effects (20). We tested the role of neuronal VEGF signaling in the structural and synaptic effects of ketamine in primary neuronal cultures and in the mPFC. We measured green fluorescent protein-labeled neuronal branching in rat primary cortical neurons 24 hours after ketamine (500 nM) or VEGF164 (50 ng/mL) incubation, with or without the selective Flk-1 inhibitor ZM323881 (10 nM, in 0.1% dimethyl sulfoxide, with 30-minute preincubation) (Figure 5A). Ketamine or VEGF164 significantly increased the number of dendrite branch crossings compared with vehicle control at both 50 μm and 100 μm from the soma, and these effects were blocked by ZM323881 (Figure 5B and 5C). For in vivo studies, control or VEGFneuron−/− mice were injected with ketamine (10 mg/kg), and then 24 hours later, brains were collected and processed for Golgi staining to visualize and analyze spine density in the apical tuft of mPFC layer V pyramidal neurons (Figure 5D and 5E). There was a small but significant decrease in spine density in the apical tuft (measured at levels I and II as indicated) in both the infralimbic and prelimbic regions of the mPFC in saline-injected VEGFneuron−/− mice compared with control mice, with the exception of the more primary tuft of the prelimbic region (level I), indicating impairment of basal synaptogenesis in the mPFC of VEGFneuron−/− mice (Figure 5F and 5G). Ketamine significantly increased spine density of layer V pyramidal neurons in both the infralimbic and prelimbic regions in control mice, but these effects were completely blocked in VEGFneuron−/− mice (Figure 5F and 5G). These results indicate that ketamine requires neuronal VEGF signaling to produce morphometric or neurotrophic and synaptogenic effects.
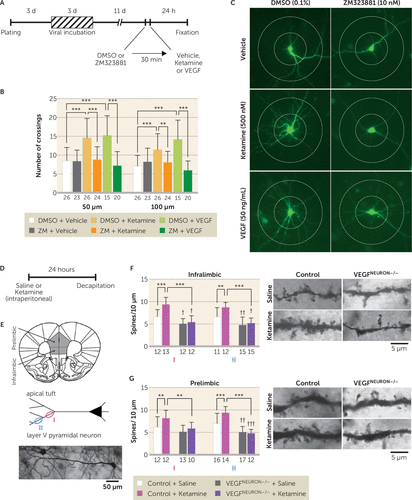
FIGURE 5. Effect of blockade of neuronal vascular endothelial growth factor (VEGF) signaling on the neurotrophic and synaptogenic effects of ketaminea
a Panel A shows the experimental timeline for dendritic morphology in rat primary cultured cortical neurons. Panel B shows the number of dendritic crossings 50 μm and 100 μm from the soma after 24-hour vehicle, ketamine (500 nM), or VEGF164 (50 ng/mL) treatment, with 30-minute preincubation of 0.1% dimethyl sulfoxide (DMSO) with or without ZM323881 (10 nM; 50 μm interaction, F=11.0, df=2, 128, p<0.0001; 100 μm interaction, F=17.2, df=2, 128, p<0.0001, N=15–26). Panel C shows representative images of expression of enhanced green fluorescent protein (EGFP) in rat primary cortical neurons from each group with concentric circles (100 μm and 200 μm in diameter). Panel D shows the experimental timeline for dendritic spine analysis. Panel E illustrates how spine density was analyzed on primary (I) and secondary (II) branches of the apical tuft of layer V pyramidal neurons in the infralimbic (11–15 branches from six cells from three mice per group) and prelimbic (10–17 branches from six cells from three mice per group) medial prefrontal cortex. At the bottom of panel E is a representative image of a Golgi-stained layer V pyramidal neuron. The left side of panel F illustrates the spine density of infralimbic pyramidal neurons (I interaction, F=8.45, df=1, 45, p=0.0056, N=12–13; II interaction, F=4.06, df=1, 49, p=0.049, N=11–15); the right side of panel F shows representative images of the apical tuft segments. The left side of panel G illustrates the spine density of prelimbic pyramidal neurons (I interaction, F=1.51, df=1, 43, p=0.23, main effect of ketamine, F=6.72, df=1, 43, p=0.013, main effect of genotype, F=10.6, df=1, 43, p=0.0022, N=10–13; II interaction, F=10.2, df=1, 55, p=0.0023, N=12–17); the right side of panel G shows representative images of the apical tuft segments. Data are expressed as means and standard deviations. d=day; h=hour; min=minute.
**p<0.01. ***p<0.001.
†p<0.05, ††p<0.01, and †††p<0.001 are relative to control plus saline mice.
Discussion
The results demonstrate that neuronal VEGF–Flk-1 signaling in the mPFC plays a pivotal role in the antidepressant-like behavioral and neurotrophic actions of ketamine. We also found that a single intra-mPFC infusion of ketamine or VEGF164 mimics the behavioral effects of systemic ketamine in a neuronal Flk-1–dependent manner. These findings indicate that neuronal VEGF–Flk-1 signaling in the mPFC is necessary and sufficient for the rapid and sustained antidepressant-like behavioral actions of ketamine. Alternative splicing of the VEGF gene results in various isoforms of different amino acid lengths, with VEGF120 and VEGF164 the most abundant (about 20% and 75%, respectively) in adult mouse brain (33). Both VEGF120 and VEGF164 bind two high-affinity tyrosine kinase receptors, Flt-1 (VEGFR1) and Flk-1 (VEGFR2), but only VEGF164 binds to the coreceptors neuropilin-1 and -2 (34). Our results demonstrate that Flk-1 is required for the behavioral actions of ketamine and VEGF164, although we cannot rule out the contribution of Flt-1 and neuropilin-1 and -2.
While VEGF–Flk-1 signaling is required for the actions of ketamine, the results demonstrate that blockade of neuronal VEGF–Flk-1 signaling did not result in increased immobility in the forced swim and tail suspension tests, increased latency to feed in the novelty-suppressed feeding test, or decreased time in the female urine sniffing test. These findings indicate that under controlled, environmental, nonstress conditions, loss of neuronal VEGF–Flk-1 signaling is not sufficient to produce these behavioral changes. Similar findings have been observed in BDNF mutant mice; only when these mice are exposed to stress is depressive vulnerability observed (35–37). This could be due to the simultaneous actions of multiple neurotrophic factors, such that loss of one (e.g., BDNF or VEGF) is insufficient to produce behavior deficits. However, a previous study reported that viral-mediated VEGF knockdown in the hippocampus increased immobility and latency to feed in the forced swim test and novelty-suppressed feeding test, respectively (38); this could be due to nonselective VEGF knockdown, in glia and endothelial cells as well as neurons, whereas deletion in the present study was specific to neurons. The earlier study also reported that nonselective VEGF knockdown in the hippocampus reduced but did not block the behavioral actions of ketamine. Further studies are needed to determine whether blockade of both VEGF and BDNF signaling in neurons and/or glial and endothelial cells in the mPFC is sufficient to produce behavior deficits. In addition, studies are needed to determine whether knockdown of neuronal VEGF–Flk-1 signaling increases susceptibility to stress in rodent models, such as chronic unpredictable stress or social defeat (21), and whether the behavioral actions of ketamine in these models require VEGF–Flk-1 signaling.
Neuron-specific deletion of Flk-1 or VEGF did not result in overt developmental phenotypes, although there were some subtle effects. Basal spine density in layer V pyramidal neurons was decreased, and open arm time in the elevated plus maze was reduced, in the VEGFneuron−/− mice; in Flk-1neuron−/− mice, preswim immobility was increased, latency to feed in the novelty-suppressed feeding test was decreased (in saline- and/or ketamine-injected mice), and center time in the open field test decreased. The mechanisms underlying these effects are unknown but may be related to neuronal Flk-1 deletion throughout the forebrain, as region-specific, viral-mediated Flk-1 knockdown in the mPFC had no effect on forced swim test or novelty-suppressed feeding behaviors. Moreover, these effects were not observed in VEGFneuron−/− mice. Further studies using viral-mediated Flk-1 knockdown are needed to determine whether other forebrain regions could explain the complex phenotypes shown in the Flk-1neuron−/− mice. Alternatively, these behavioral differences could be related to the housing conditions: single-housed Flk-1flox/flox/Flk-1neuron−/− mice were more active than VEGFflox/flox/VEGFneuron−/− mice in the locomotor activity and tail suspension test, but there was no difference in locomotor activity when these lines were group housed. Also, although Flk-1flox/flox and VEGFflox/flox mice were backcrossed onto C57BL/6J mice, there could still be genetic variation that could account for the locomotor differences. Notably, the Flk-1flox/flox line includes background of the outbred ICR strain (26) that shows higher locomotor activity in a novel environment than C57BL/6J mice (39). Despite these baseline differences, the controls for both lines show responses to ketamine that are blocked in the littermate knockout mice, demonstrating the utility of these lines for our studies of neuronal VEGF and Flk-1. Taken together, the conditional, cell-specific knockout approach provides strong evidence that forebrain excitatory neurons are both the source and the target of VEGF that is released in response to ketamine.
Analysis of c-Fos shows that VEGF-dependent neuronal activation in the mPFC is associated with the behavioral actions of ketamine. This is consistent with our previous results demonstrating that neuronal silencing of the infralimbic mPFC blocks the induction of c-Fos and the behavioral effects of ketamine and that in vivo optogenetic stimulation of the mPFC produces ketamine-like behavioral responses in the forced swim test, novelty-suppressed feeding test, and sucrose preference test (a test for reward or anhedonia) in rats (30). Consistent with these data, the present study shows that infusion of a VEGF neutralizing antibody into the mPFC prior to ketamine administration completely suppresses both the behavioral responses and c-Fos. In contrast, infusion of the VEGF neutralizing antibody into the mPFC 2 hours after ketamine administration did not block the behavioral effects of ketamine. Moreover, ketamine did not alter VEGF levels in the PFC within 1 hour. Taken together, the results indicate that ketamine rapidly and transiently increases VEGF release, but not protein levels, in the mPFC and that VEGF–Flk-1 signaling during the initial 2-hour period is required for the rapid and sustained behavioral actions of ketamine (Figure 6). Previous studies report that ketamine increases levels of VEGF as well as BDNF in the hippocampus, but these studies did not test whether hippocampal VEGF or BDNF release is required for the actions of ketamine (38, 40), as was done in the present study and in our previous work investigating BDNF (22, 23). These findings indicate that rapid increases in VEGF and BNDF release, but not expression, in the mPFC at early time points less than 2 hours postinjection are critical for the behavioral effects of ketamine.
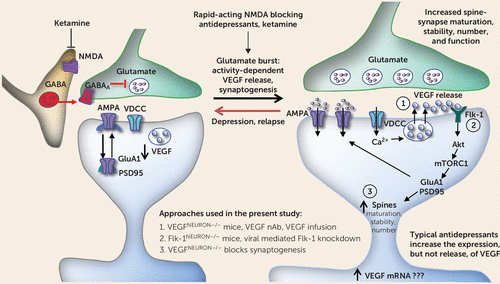
FIGURE 6. Regulation of vascular endothelial growth factor (VEGF) release and Flk-1 signaling in a model for the cellular actions of ketaminea
a Ketamine blockade of N-methyl-d-aspartate (NMDA) receptors located on GABA-ergic interneurons results in disinhibition and a rapid but transient glutamate burst that activates AMPA receptors. This leads to stimulation of l-type voltage-dependent calcium channels (VDCCs) and influx of Ca2+ that stimulates VEGF release, which stimulates Flk-1 signaling, including activation of the mechanistic target of rapamycin complex 1 (mTORC1) pathway. The mTORC1 pathway controls the translation and synthesis of synaptic proteins, including GluA1, a subtype of the AMPA receptor, and postsynaptic density protein 95 (PSD95), that are required for increases in synaptogenesis and spine maturation. These cellular events are associated with the rapid and sustained antidepressant actions of ketamine. Previous studies have demonstrated that release of brain-derived neurotrophic factor also plays an essential role in the rapid and sustained actions of ketamine (see the Discussion section). Akt=a serine/threonine protein kinase; nAb=neutralizing antibody.
Previous studies have demonstrated that ketamine rapidly increases the number and function of spine synapses on layer V pyramidal neurons in the mPFC through activity-dependent BDNF release and activation of the mechanistic target of rapamycin complex 1 (mTORC1) (5, 20, 23). The present results demonstrate that VEGF–Flk-1 signaling is sufficient to increase dendrite complexity in primary neurons, consistent with a previous report (24). VEGF–Flk-1 is also necessary for ketamine induction of dendrite complexity and spine synapse number in layer V mPFC pyramidal neurons, as these effects were blocked by pharmacological inhibition of Flk-1 and in VEGFneuron−/− mice, respectively. These results indicate that neuronal VEGF, as well as BDNF (22, 23) signaling, play key roles in the synaptogenic effects of ketamine. In addition, spine density is decreased in the mPFC of VEGFneuron−/− mice, similar to what is seen with chronic stress (21, 41, 42), although these mice do not display behavior deficits in models of despair, anxiety, and motivation or reward. Similar morphological, but not behavioral, deficits (i.e., despair in the forced swim test) have been reported in BDNF Val66Met knock-in mice in which the secretion of BDNF is impaired (23). These findings suggest that reductions of both VEGF and BDNF signaling are required to produce behavior deficits, as deletion or inhibition of only one pathway is insufficient. Whether these trophic factors act in parallel or sequentially remains to be determined. BDNF is reported to stimulate VEGF expression via mTORC1 in neuroblastoma cells, supporting the possibility that VEGF–Flk-1 signaling is downstream of BDNF (43). However, it is also possible that ketamine induction of neuronal activity causes release of both BDNF and VEGF that then act simultaneously.
In summary, the present findings demonstrate that VEGF signaling in the mPFC plays an essential role in the behavioral and synaptic effects of ketamine and may have important implications for understanding the pathophysiology and treatment of depression. Although the role of VEGF in depression remains unknown, a recent genome-wide association study revealed that a VEGF-related single-nucleotide polymorphism (SNP), rs4416670, is associated with increased risk for depression (44), and the minor C allele of this SNP is associated with decreased levels of VEGF (45). Together, these findings raise the possibility that patients with the C allele and reduced levels of VEGF would not respond to ketamine to the same extent as patients with the major T allele SNP. Further studies of the impact of this SNP in humans as well as in rodent models could test this interesting hypothesis. In addition, the present findings could help identify novel VEGF–Flk-1 signaling sites to target for the development of new, rapid-acting antidepressants.
1 World Health Organization: Depression fact sheet, 2018. http://www.who.int/news-room/fact-sheets/detail/depressionGoogle Scholar
2 : The economic burden of adults with major depressive disorder in the United States (2005 and 2010). J Clin Psychiatry 2015; 76:155–162Crossref, Medline, Google Scholar
3 : Subgenual prefrontal cortex abnormalities in mood disorders. Nature 1997; 386:824–827Crossref, Medline, Google Scholar
4 : Common and distinct patterns of grey-matter volume alteration in major depression and bipolar disorder: evidence from voxel-based meta-analysis. Mol Psychiatry 2017; 22:1455–1463Crossref, Medline, Google Scholar
5 : Synaptic plasticity and depression: new insights from stress and rapid-acting antidepressants. Nat Med 2016; 22:238–249Crossref, Medline, Google Scholar
6 : Morphometric evidence for neuronal and glial prefrontal cell pathology in major depression. Biol Psychiatry 1999; 45:1085–1098Crossref, Medline, Google Scholar
7 : A neurotrophic model for stress-related mood disorders. Biol Psychiatry 2006; 59:1116–1127Crossref, Medline, Google Scholar
8 : Neurotrophin levels in postmortem brains of suicide victims and the effects of antemortem diagnosis and psychotropic drugs. Brain Res Mol Brain Res 2005; 136:29–37Crossref, Medline, Google Scholar
9 : Abnormal retinoid and TrkB signaling in the prefrontal cortex in mood disorders. Cereb Cortex 2015; 25:75–83Crossref, Medline, Google Scholar
10 : Differential brain, but not serum VEGF levels in a genetic rat model of depression. Neurosci Lett 2010; 474:13–16Crossref, Medline, Google Scholar
11 : Chronic stress in the adult dentate gyrus reduces cell proliferation near the vasculature and VEGF and Flk-1 protein expression. Eur J Neurosci 2005; 21:1304–1314Crossref, Medline, Google Scholar
12 : Low vascular endothelial growth factor and interleukin-8 in cerebrospinal fluid of suicide attempters. Transl Psychiatry 2012; 2:e196Crossref, Medline, Google Scholar
13 : Essential role of brain-derived neurotrophic factor in adult hippocampal function. Proc Natl Acad Sci USA 2004; 101:10827–10832Crossref, Medline, Google Scholar
14 : Regulation of BDNF and trkB mRNA in rat brain by chronic electroconvulsive seizure and antidepressant drug treatments. J Neurosci 1995; 15:7539–7547Crossref, Medline, Google Scholar
15 : Chronic antidepressant administration increases the expression of cAMP response element binding protein (CREB) in rat hippocampus. J Neurosci 1996; 16:2365–2372Crossref, Medline, Google Scholar
16 : Activation of the TrkB neurotrophin receptor is induced by antidepressant drugs and is required for antidepressant-induced behavioral effects. J Neurosci 2003; 23:349–357Crossref, Medline, Google Scholar
17 : VEGF is an essential mediator of the neurogenic and behavioral actions of antidepressants. Proc Natl Acad Sci USA 2007; 104:4647–4652Crossref, Medline, Google Scholar
18 : Vascular endothelial growth factor signaling is required for the behavioral actions of antidepressant treatment: pharmacological and cellular characterization. Neuropsychopharmacology 2009; 34:2459–2468Crossref, Medline, Google Scholar
19 : A randomized trial of an N-methyl-d-aspartate antagonist in treatment-resistant major depression. Arch Gen Psychiatry 2006; 63:856–864Crossref, Medline, Google Scholar
20 : mTOR-dependent synapse formation underlies the rapid antidepressant effects of NMDA antagonists. Science 2010; 329:959–964Crossref, Medline, Google Scholar
21 : Glutamate N-methyl-d-aspartate receptor antagonists rapidly reverse behavioral and synaptic deficits caused by chronic stress exposure. Biol Psychiatry 2011; 69:754–761Crossref, Medline, Google Scholar
22 : BDNF release is required for the behavioral actions of ketamine. Int J Neuropsychopharmacol 2014; 18:pyu033Crossref, Medline, Google Scholar
23 : Brain-derived neurotrophic factor Val66Met allele impairs basal and ketamine-stimulated synaptogenesis in prefrontal cortex. Biol Psychiatry 2012; 71:996–1005Crossref, Medline, Google Scholar
24 : Neurotrophic effects of vascular endothelial growth factor on organotypic cortical explants and primary cortical neurons. J Neurosci 2003; 23:11036–11044Crossref, Medline, Google Scholar
25 : A CamKIIalpha iCre BAC allows brain-specific gene inactivation. Genesis 2001; 31:37–42Crossref, Medline, Google Scholar
26 : Alternatively spliced vascular endothelial growth factor receptor-2 is an essential endogenous inhibitor of lymphatic vessel growth. Nat Med 2009; 15:1023–1030Crossref, Medline, Google Scholar
27 : VEGF is required for growth and survival in neonatal mice. Development 1999; 126:1149–1159Medline, Google Scholar
28 : GABA interneurons mediate the rapid antidepressant-like effects of scopolamine. J Clin Invest 2016; 126:2482–2494Crossref, Medline, Google Scholar
29 : High-fat diet induced anxiety and anhedonia: impact on brain homeostasis and inflammation. Neuropsychopharmacology 2016; 41:1874–1887Crossref, Medline, Google Scholar
30 : Optogenetic stimulation of infralimbic PFC reproduces ketamine’s rapid and sustained antidepressant actions. Proc Natl Acad Sci USA 2015; 112:8106–8111Crossref, Medline, Google Scholar
31 : REDD1 is essential for stress-induced synaptic loss and depressive behavior. Nat Med 2014; 20:531–535Crossref, Medline, Google Scholar
32 : Ribosomal protein S6 kinase 1 signaling in prefrontal cortex controls depressive behavior. Proc Natl Acad Sci USA 2015; 112:6188–6193Crossref, Medline, Google Scholar
33 : Differential expression of VEGF isoforms in mouse during development and in the adult. Dev Dyn 2001; 220:112–121Crossref, Medline, Google Scholar
34 : Vascular endothelial growth factor (VEGF) and its receptors. FASEB J 1999; 13:9–22Crossref, Medline, Google Scholar
35 : Gender differences in the enhanced vulnerability of BDNF+/– mice to mild stress. Int J Neuropsychopharmacol 2009; 12:583–588Crossref, Medline, Google Scholar
36 : A role for MAP kinase signaling in behavioral models of depression and antidepressant treatment. Biol Psychiatry 2007; 61:661–670Crossref, Medline, Google Scholar
37 : Variant brain-derived neurotrophic factor Val66Met polymorphism alters vulnerability to stress and response to antidepressants. J Neurosci 2012; 32:4092–4101Crossref, Medline, Google Scholar
38 : Hippocampal VEGF is necessary for antidepressant-like behaviors but not sufficient for antidepressant-like effects of ketamine in rats. Biochim Biophys Acta 2016; 1862:1247–1254Crossref, Medline, Google Scholar
39 : Manipulation of dopamine metabolism contributes to attenuating innate high locomotor activity in ICR mice. Behav Brain Res 2017; 328:227–234Crossref, Medline, Google Scholar
40 : NMDA receptor blockade at rest triggers rapid behavioural antidepressant responses. Nature 2011; 475:91–95Crossref, Medline, Google Scholar
41 : The mood stabilizer lithium potentiates the antidepressant-like effects and ameliorates oxidative stress induced by acute ketamine in a mouse model of stress. Int J Neuropsychopharmacol 2014; 18:pyu102Medline, Google Scholar
42 : Stress blunts serotonin- and hypocretin-evoked EPSCs in prefrontal cortex: role of corticosterone-mediated apical dendritic atrophy. Proc Natl Acad Sci USA 2008; 105:359–364Crossref, Medline, Google Scholar
43 : Brain-derived neurotrophic factor activation of TrkB induces vascular endothelial growth factor expression via hypoxia-inducible factor-1alpha in neuroblastoma cells. Cancer Res 2006; 66:4249–4255Crossref, Medline, Google Scholar
44 : VEGF-related polymorphisms identified by GWAS and risk for major depression. Transl Psychiatry 2017; 7:e1055Crossref, Medline, Google Scholar
45 : Identification of cis- and trans-acting genetic variants explaining up to half the variation in circulating vascular endothelial growth factor levels. Circ Res 2011; 109:554–563Crossref, Medline, Google Scholar