An fMRI Auditory Oddball Study of Combined-Subtype Attention Deficit Hyperactivity Disorder
Abstract
Objective: Studies of attention deficit hyperactivity disorder (ADHD) have reliably found reduced amplitude event-related potentials (ERPs) measuring attention-related brain function, indicating impairment in the brain’s ability to automatically orient attention to odd or novel environmental stimuli and to represent that information in working memory. However, the relationship between abnormal neurocognition and dysfunction in specific brain regions in ADHD remains unclear. Method: The authors used functional magnetic resonance imaging (fMRI) to identify brain regions with abnormal hemodynamic activity during processing of target and novelty oddball stimuli that engage attention. Forty-six boys 11–18 years of age participated in the study, including 23 diagnosed as having ADHD with hyperactivity and impulsivity (combined type) and 23 demographically matched control subjects. Event-related fMRI data were collected while participants performed a three-stimulus auditory oddball task. Hemodynamic activity was compared between ADHD participants and control subjects in brain regions previously linked to P3 ERPs. Results: Participants with ADHD showed deficits in brain activity elicited by infrequent attentionally engaging stimuli in regions associated with attentional orienting and working-memory cognitive processes. These deficits co-occurred with highly variable and slow task performance. Conclusions: This study links ADHD attentional orienting and working-memory deficits to dysfunction in specific cortical brain regions. The results indicate that ADHD pathophysiology impairs brain systems that are important for allocating attention and using cognitive representations to guide cognition and behavior. Attention-related neural dysfunction is thus an important factor to consider in neurobiological theories of ADHD.
A diagnosis of attention deficit hyperactivity disorder (ADHD) is given to children and adults who have developmentally inappropriate impulsive, hyperactive, or inattentive behavior severe enough to impair social, educational, or occupational activities (1) . Studies of neuropsychological performance (2 , 3) , brain function (4 , 5) , brain structure (6) , and molecular genetics (7) indicate that ADHD has a neurobiological basis. Theories propose that ADHD is associated with impairment in executive functions that regulate or direct attention and behavioral control (2 , 3 , 8 , 9) . A consistently replicated neurocognitive abnormality in ADHD is reduction of event-related potentials (ERPs) during performance of attentionally demanding tasks requiring detection of infrequent target stimuli (that is, “oddballs”; see review in reference 4 ). It has been argued that low-probability oddball stimuli engage orienting of attention (10) , as reflected by ERPs peaking ∼300 msec after infrequent stimuli—the P3a and P3b. The P3a is believed to represent orienting of attention to rare event types (11) ; it also follows unique and engaging novel sounds (i.e., “novelty P3”) (12 – 14) . The P3b follows task-relevant target stimuli (15) , peaks slightly later than the P3a, has a more posterior scalp topography, and is thought to index decision-making processes and contextual updating of working memory (16) . Intracranial recordings indicate that P3a reflects activity in the inferior frontal sulcus, superior temporal plane, supramarginal gyri, and anterior cingulate cortex (11 , 17) . Target P3b is associated with activity in the hippocampus, superior temporal sulcus, ventrolateral prefrontal cortex, anterobasal temporal lobe, and superior parietal lobule (11 , 17 – 19) . These intracranial results have been confirmed by numerous functional magnetic resonance imaging (fMRI) experiments (20 – 32) . Target P3b links to temporal-parietal brain activity are supported and extended by EEG spatiotemporal modeling using fMRI data dipole sources (31 – 33) and EEG/fMRI fusion (34) .
Children with ADHD consistently show reduced posterior brain target P3b amplitude on oddball tasks requiring a goal-directed response (35 – 41) . Although several studies provide contradictory evidence (42 – 44) , evidence from larger, well-controlled studies indicates that children and adolescents with ADHD show abnormal target P3b amplitude (37 , 41) . Contradictory evidence has come only from samples with mixed DSM-IV subtypes of ADHD (42) , samples with comorbid psychiatric conditions, and samples for which comorbidity is not reported (42 – 44) . Some studies have also found reduced P3a elicited by oddball “novel” stimuli in youths with ADHD (35 , 39) . There is mixed evidence for other ERP abnormalities in ADHD. The largest studies with rigorously controlled sampling criteria found reduced P1, N1, P2, and N2 amplitudes in youths with ADHD (37 , 39 , 41) .
This study was motivated by the need to identify the neural substrates of attentional orienting dysfunction in ADHD. To date, only one study has examined oddball-elicited brain function in ADHD. Tamm and colleagues (45) found diminished hemodynamic activity in ADHD subjects in the parietal lobe bilaterally, the thalamus, and the mid-cingulate regions compared with healthy control subjects on a choice visual oddball task. The Tamm et al. study did not examine the brain response to novelty stimuli, which more aptly reflects automatic attentional orienting. In addition, the brain’s response to infrequent target stimuli is believed to engage cognitive processes different from novelty stimuli processing. The “attention-grabbing” nature of novelty stimuli make them particularly relevant to the study of ADHD, a disorder in which distractibility is a central characteristic. We used an event-related fMRI auditory oddball task to examine hemodynamic activity in ADHD in brain regions linked to P3 ERPs to both target and novelty stimuli, thus extending the results previously reported by Tamm et al. (45) . We chose to focus on adolescents with ADHD with hyperactivity and impulsivity (ADHD combined type, DSM-IV diagnostic code 314.01) because previous studies (35 , 37) found ERP differences between DSM-IV ADHD subtypes on this paradigm. Hereafter in this article, “ADHD” refers only to DSM-IV 314.01 diagnoses.
Hypotheses were based on the prediction that adolescents with ADHD would have deficits in automatic attentional orienting (to both target and novel stimuli) and working-memory contextual updating processes (during target stimuli processing). Based on intracranial recording and ERP/fMRI fusion work cited above, adolescents with ADHD were hypothesized to have less hemodynamic activity in response to target stimuli than control adolescents in the superior temporal sulcus regions, the middle/inferior frontal gyri, the anterobasal temporal lobe, the inferior parietal lobule, and the superior parietal lobule. Consistent with putative novelty-P3a neural generators, we predicted that adolescents with ADHD would show less activity in response to novel stimuli in the inferior frontal sulcus region, the superior temporal plane, the left and right supramarginal gyri, and the anterior cingulate cortex. These predictions also are consistent with the few previous fMRI studies of ADHD showing abnormal activity in the anterior cingulate (46–49) and lateral prefrontal cortex regions (47, 48, 50).
Method
Participants
Participants were 46 boys 11–18 years of age; 23 had been diagnosed as having ADHD and had lifetime psychostimulant treatment histories, and 23 were demographically matched healthy control subjects who were recruited via physician referral and community advertisements. ADHD diagnosis was evaluated by the Schedule for Affective Disorders and Schizophrenia for School-Age Children—Present and Lifetime Version (51) administered by experienced staff. No participant had any non-ADHD psychiatric diagnoses, history of formal learning disability, or significant medical conditions. Because two participants with ADHD were left-handed by self-report, a similar number of left-handed participants, matched by age, were included in the control group. Study group characteristics and t test comparison results are reported in Table 1 . Study groups were matched on age, socioeconomic status (52) , self-report scores for depression (53) and anxiety (54) , and estimated intelligence (55) . Neuropsychological impairment was assessed with the Conners Continuous Performance Test—2nd ed. (56) . The symptoms of all but one ADHD participant were managed by psychostimulant medication; 18 were taking sustained-release/long-acting methylphenidate; one was taking a twice-daily formulation of methylphenidate; one was taking both sustained-release/long-acting methylphenidate and a once-a-day formulation of methylphenidate; and three were taking extended-release mixed amphetamine salts. At the time of fMRI, ADHD participants had not taken medication for at least 24 hours.
Study procedures were explained in detail to potential participants and a parent or legal guardian in the course of an informed consent/assent protocol approved by Hartford Hospital’s institutional review board. Research procedures adhered to ethical standards. Study participants received monetary compensation for their time.
fMRI Task and Procedure
The task was a three-stimulus auditory oddball task similar to that used in previous fMRI studies (24 – 26 , 57 , 58) , using custom software (VAPP; http://www.nrc-iol.org/vapp/) for presentation. The standard stimulus was a 1,000 Hz tone (probability=0.80), the target stimulus was a 1,500 Hz tone (probability=0.10), and the novel stimuli (probability=0.10) consisted of nonrepeating random digital noises (e.g., tone sweeps, whistles). In each run, there were 24 target tone stimuli, 24 novel sound stimuli, and 196 nontarget tone stimuli. Stimuli were presented for 200 msec with a pseudorandom stimulus onset asynchrony ranging from 1,000 to 3,000 msec (mean=1,500). Target and novel stimuli were always preceded by at least three standard stimuli and as many as five. Participants were instructed to respond as quickly and as accurately as possible with a right index finger button press for every target tone, but not for other stimuli. During scanning, all participants reported that they could hear the stimuli and discriminate them from the background scanner noise. Stimulus events and behavioral responses (hits or false alarms within 1,250 msec) were recorded and monitored online on a separate computer.
Analysis of Behavioral Performance
Two-sample t tests were used to evaluate mean reaction time for correct target responses and false alarm rates for novel and standard stimuli.
Imaging Parameters
Imaging was done on a Siemens Allegra 3T system at the Olin Neuropsychiatry Research Center of the Institute of Living/Hartford Hospital in Hartford, Conn. Functional image volumes were collected in axial orientation to the anterior commissure-posterior commissure line using a gradient-echo sequence sensitive to the blood-oxygen-level-dependent (BOLD) signal (repetition time=1,500 msec, echo time=28 msec, flip angle=65°, field of view=24×24 cm, 64×64 matrix, 3.4×3.4 mm in plane resolution, 5 mm slice thickness, 30 slices) effectively covering the entire brain (150 mm) in 1.5 sec. The two runs each consisted of 255 time points, including a 9 sec rest session at the beginning that was collected to allow T 1 effects to stabilize. These initial six images were not included in subsequent analyses.
Image Processing
Functional images were reconstructed offline, and each run was separately realigned using INRIAlign (59 , 60) as implemented in the Statistical Parametric Mapping software (SPM2, Wellcome Department of Cognitive Neurology, London). Two-sample t tests found group differences in one of six realignment coefficients: we found evidence, at an uncorrected p value <0.05, that pitch rotations were greater in adolescents with ADHD (see Table 1 ). The fact that one of these six motion-correction parameters showed group differences raises the possibility that motion could influence group comparisons of brain activation. In addition, 12 participants in the entire sample (eight in the ADHD group and four in the control group) had displacement >1 voxel length. Although a valid case could be made for the conservative approach of excluding these cases from the analysis, we instead assessed the impact of motion on our data to determine empirically whether the larger sample should be retained. First, we compared the magnitude of left postcentral gyrus activation during target detection in participants with >1 voxel movement and those with less. Because signal strength in the motor cortex would not be expected to differ between groups on this task, a direct comparison between participants with more versus less movement serves as an internal quality control. In an 8 mm radius around voxel coordinates reported in previous work (26) (x,y,z=–57, 21, 18), no group differences were observed. Thus, there was no evidence that the group difference in mean pitch movements or that the handful of participants with head displacement >1 voxel length adversely affected activation amplitude. We found no evidence that head motion in either group was correlated with onsets of target or novel stimuli. Also, no significant group differences in correlation coefficients were found, indicating that motion did not differentially affect a condition of interest in either group. Collectively, these data strongly suggest that there was no adverse impact of head movement on BOLD signal. Study hypotheses were evaluated using the full sample; however, the same analyses using the subsample (N=34) with low movement is also presented to provide confidence in the results.
A mean functional image volume was constructed for each participant for each session from the realigned image volumes and was used to determine parameters for spatial normalization into standardized Montreal Neurological Institute space. These normalization parameters were then applied to the corresponding functional image volumes. Normalized images were smoothed with a 12 mm 3 full width at half maximum Gaussian filter. A fifth-order IIR Butterworth low-pass filter of 0.16 Hz removed high-frequency noise associated with alterations in the applied radio frequency field.
fMRI Statistics
The regressors for each participant’s fMRI model were derived by extracting stimulus onset timing for correct target, novel, and standard stimuli and modeled using a synthetic hemodynamic response function and temporal derivative (61) . Six motion-correction parameter estimates (x, y, and z displacement and roll, pitch, and yaw rotations) were included as covariates of no interest to statistically control signal change related to motion. A high-pass filter (cutoff period=128 sec) was incorporated into the model to remove low-frequency signals. For each condition of interest, SPM2 wrote an image in which each voxel represented the estimated amplitude of hemodynamic response. A latency variation amplitude-correction method provided a more accurate estimate of hemodynamic response for each condition (62) . Group analyses used these latency-corrected contrast images in random-effects statistical analyses.
Group Analyses
First, we verified that participants in both the ADHD group and the healthy control group showed activity in the full set of regions of interest (ROIs) identified in our previous reports (25 , 26) . ROIs were voxel clusters of 2.187 cm 3 (8 mm radius spheres) centered at locations of target-elicited hemodynamic activity (37 ROIs), novel-elicited activity (31 ROIs), and standard-elicited activity (4 ROIs) from previous work (25 , 26) . One target-processing ROI in the left postcentral gyrus and two novelty-processing ROIs in the cerebellum were omitted because they were too proximal to others. Because there were no group differences in any standard-stimulus-processing ROIs (26) , we contrasted hemodynamic activity elicited by target and novel stimuli relative to a baseline produced by frequent standards, as in our previous reports (24 , 26) . We used random-effects one-sample t tests separately for ADHD and control participants to determine whether there was activity in all a priori defined target- and novelty-processing ROIs. Bonferroni corrections were used to adjust for multiple statistical tests. For 37 target-processing ROIs, p<0.05/37=0.0014 (t critical =3.40). For the 31 novelty-processing ROIs, p<0.05/31=0.0016 (t critical =3.31). Results are reported as the maximum t score within each ROI.
Second, we contrasted brain activity in ADHD and control participants in a smaller subset of ROIs suggested by the theoretical and empirical research reviewed above. Hypotheses were addressed with random-effects two-sample t tests examining the 12 target-processing ROIs and nine novelty-processing ROIs predicted a priori to differ between groups. For target-processing ROIs, group differences were examined in the left middle frontal gyrus, left and right inferior frontal gyri, anterior cingulate, left and right inferior parietal lobules/supramarginal gyri, left and right superior parietal lobules, and left and right superior and middle temporal gyri. For novel stimuli, ROIs were examined in the left and right middle/inferior frontal gyri, anterior cingulate, left inferior parietal lobule/supramarginal gyrus, and left and right posterior superior temporal gyri. Bonferroni-corrected thresholds were an alpha of 0.004167 (0.05/12) and a t critical =2.77 for target stimuli, and an alpha of 0.0056 (0.05/9) and a t critical =2.65 for novel stimuli. We also conducted supplemental exploratory analyses using statistical corrections for searching the whole brain to determine whether any group differences fell outside these ROIs (using a liberal threshold of p<0.05 uncorrected). The text and tables differentiate findings for hypothesized regions (i.e., Bonferroni-corrected, p Bonf ) and exploratory (uncorrected, p uncorr ) findings. Results of group analyses are displayed using cortical surface renderings with hemodynamic activity overlays (63) . Finally, to address any remaining possibility that head movements in several participants may have influenced the results, we reexamined the subsample whose head displacement was ≤1 voxel length in either fMRI run. Because the smaller sample size provided less statistical power, we assessed this evidence at a p uncorr of <0.05 and found that it indicated the same effect as in the larger sample. Because the sample size was smaller, the critical t statistics for target and novel stimuli were adjusted for degrees of freedom, yielding t=2.82 and t=2.69, respectively.
Results
Behavioral Performance
There were no statistically significant differences between participants in the ADHD and healthy control groups in the number of hits or false alarms in response to novel or standard stimuli ( Table 1 ). Participants with ADHD were slower than control participants to respond to target stimuli (t=1.839, df=44, p=0.073).
Main Effect of Target and Novel Stimuli in ADHD and Healthy Adolescents
In healthy adolescents, there was significant activity in 36 of the 37 target-processing ROIs ( Table 2 ) found active in previous studies and 23 of the 31 novelty-processing ROIs ( Table 3 ). For target stimuli, only the right superior parietal lobule ROI did not show suprathreshold activity at p Bonf <0.05; however, this region did show activity when a lower statistical threshold (p uncorr <0.01) was used. Target detection elicited significant hemodynamic activity in 36 of the 37 ROIs for ADHD participants at p Bonf <0.05. As with healthy adolescents, evidence for target activity in the right superior parietal lobule only reached trend levels (p uncorr <0.01). For novel stimuli, only 13 of the 31 ROIs (p Bonf <0.05) were engaged in ADHD participants. When a liberal threshold was used (i.e., p uncorr <0.05 or <0.01), there was evidence that seven additional ROIs were engaged in ADHD participants during novelty processing. ADHD participants failed to engage the remaining 11 novelty-processing ROIs ( Table 3 ). Thus, healthy control participants activated almost all target- and novelty-processing ROIs. Participants in the ADHD group activated almost all target-processing ROIs but less than half of the novelty-processing ROIs identified in previous research. Supplemental analysis did not find any activation to target or novel stimuli outside ROIs at any statistical threshold.
Between-Groups Comparisons of Hemodynamic Response to Target and Novel Stimuli
Results for the comparison of ADHD and control groups on response to target stimuli are shown in Table 2 and illustrated in Figure 1 . Consistent with our hypotheses, ADHD participants had less hemodynamic activity than healthy controls in the left middle frontal gyrus, the anterior regions of the right superior temporal gyrus, and the middle region of the right middle temporal gyrus (p Bonf <0.05). For several other hypothesized brain regions, there was evidence at lower statistical thresholds (p uncorr <0.05) for less ADHD neural activity to target stimuli ( Table 2 ). There were no group differences in the superior parietal lobules for target detection (p Bonf <0.05 or p uncorr <0.05).
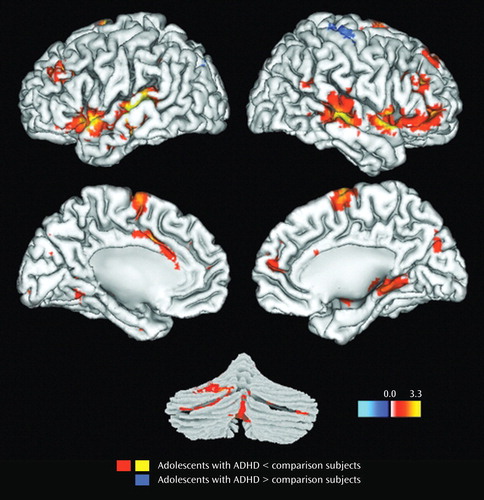
a Statistical results are thresholded at a low of p<0.05 (uncorrected) and a high of p<0.001 (uncorrected). Yellow-orange indicates regions where amplitude is lower in the ADHD group than in the control group. Blue indicates regions where amplitude is higher in the ADHD group than in the control group.
Results for the comparison of ADHD and control groups on response to novel stimuli are shown in Table 3 and illustrated Figure 2 . In brain regions predicted a priori to differ between groups, participants with ADHD showed diminished hemodynamic activity in response to novel stimuli in the left inferior parietal lobule/supramarginal gyrus and the posterior region of the left superior temporal gyrus. For several other hypothesized brain regions, evidence for group differences in activation was found only when liberal statistical thresholds were used (p uncorr <0.05; see Table 3 ). There was no evidence for hypothesized group differences in the left or right anterior cingulate for novelty processing.
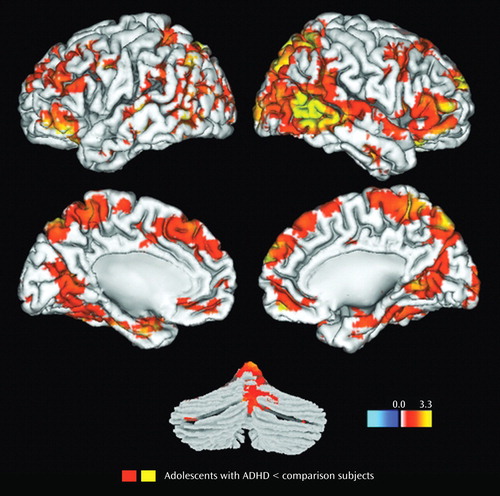
a Statistical results are thresholded at a low of p<0.05 (uncorrected) and a high of p<0.001 (uncorrected). Yellow-orange indicates regions where amplitude is lower in the ADHD group than in the control group.
In no ROI did participants with ADHD show a greater amplitude of hemodynamic response relative to control subjects for either target or novel stimuli. Supplemental analysis did not find any group differences outside the ROIs using statistical thresholds appropriate for searching the entire brain.
The reexamination of data using participants whose head motion did not exceed 1 voxel length (N=15 for the ADHD group and N=19 for the control group) supported nearly all these results, albeit not using stringent Bonferroni corrections for searching multiple ROIs. For brain regions hypothesized to differ between groups during target processing, t tests failed to reach the critical t statistic (at p uncorr <0.05) in peak voxels only for the ROIs in the right inferior frontal gyrus, the anterior cingulate, and the left middle temporal gyrus. All other target effects were validated. For novel stimuli, the reanalysis of the smaller subsample did not find group differences in the right middle/inferior frontal gyrus and the right posterior superior temporal gyrus or the left superior/middle frontal gyrus (although the latter region approached significance). In two novelty-processing ROIs, the peak group difference was validated but was found in a different voxel within each ROI (left middle/inferior frontal gyrus [x,y,z=–51, 3, 36; t=1.88, df=32, p uncorr <0.05] and left superior/middle temporal gyrus [x,y,z=–60, –18, –9; t=1.31, df=32, p uncorr <0.05]).
Discussion
We compared hemodynamic activity elicited in adolescents with ADHD and demographically matched healthy control subjects during oddball stimulus processing to identify the neural substrates of attentional dysfunction in ADHD. Consistent with our hypotheses, participants with ADHD had less hemodynamic activity than control subjects during target processing in the left middle frontal gyrus and the right superior temporal gyrus. To novel stimuli, participants with ADHD had hemodynamic deficits in the left inferior parietal lobule/supramarginal gyrus and the posterior region of the left superior temporal gyrus. Hemodynamic deficits to both target and novel stimuli in ADHD occur in brain regions linked to automatic attentional orienting and the P3a in previous electrophysiology studies (11 , 17) . Deficits in the right middle frontal gyrus and anterior right superior temporal gyrus are regions linked to P3b (11 , 17 – 19) . The P3b is generally believed to reflect cognitive processes involved with contextual updating of working memory (16) . Thus, our results in this study provide evidence that ADHD deficits in attentional orienting and the ability to represent task-relevant target stimuli in working memory are linked to several specific frontal, temporal, and parietal lobe regions. These regions are part of a dopaminergically innervated system implicated as hypofunctional in ADHD by neuropsychological (64) , brain structural (6) , neurochemical (65) , and functional neuroimaging evidence (5) . On the Conners Continuous Performance Test, adolescents in the ADHD group were impaired in their ability to detect target stimuli and had highly variable performance, suggesting clinically significant impairment consistent with that identified in previous ADHD research (3 , 66 , 67) . This result, along with the statistical trend toward longer target reaction time to target stimuli in ADHD, indicates that these regional brain function abnormalities likely underlie slow stimulus evaluation or subsequent impaired decision-making cognitive processes. Therefore, our results link ADHD attention-related cognitive impairment to cortical dysfunction within an abnormally functioning frontostriatal system.
These results are consistent with results reported by Tamm et al. (45) , who found evidence for parietal lobe dysfunction on an oddball task in ADHD. However, we also found evidence for a greater number of dysfunctional ADHD brain regions than did Tamm et al. These differences are likely related to the greater statistical power from the larger sample size in this study and possibly to the different sensory modality used (visual versus auditory; see reference 25 ) or use of proportional scaling by Tamm et al. (45) . Proportional scaling can produce artifactual deactivations in BOLD fMRI when the local signal (e.g., to frequent target stimuli) contributes greatly to the global signal, as it does in oddball tasks (68) . It also is possible that differences between the two studies reflect neurobiological heterogeneity in ADHD (see references 69 and 70 for discussions of single- versus multiple-effects models of ADHD). Indeed, the observation of generally low t scores for all group differences and the fact that some statistically significant effects were not replicated even at liberal statistical thresholds in the low-movement subsample raises the possibility that there may be heterogeneity of brain activation within the ADHD group. Our results ultimately may direct future research in seeking biological markers consistent with an etiological factor specific to attentional dysfunction that is perhaps present in only a subgroup of persons with ADHD. Our study extends the results of Tamm et al. by reporting ADHD versus non-ADHD differences in brain activation in response to novelty oddball stimuli. Because novelty stimuli are believed to more directly engage attentional orienting cognitive processes relative to target detection, the evidence for impaired parietal and temporal brain region activation in ADHD further links these cortical regions to ADHD pathophysiology underlying allocation of attentional resources. Our findings also are consistent with previous fMRI studies of ADHD that found abnormalities in the lateral prefrontal cortex (47 , 48 , 50) . There were no group differences in brain regions that were not expected to vary on this task (i.e., the primary motor cortex during target responses), which supports the specificity of these results both to ADHD and to attentional dysfunction.
ADHD neurobiological theories variously propose core impairments in response inhibition (71 , 72) , other executive functions needed for attentional or behavioral control (2 , 3 , 9) or motivation or effort (69 , 73) . Our findings cannot easily be attributed to impaired response inhibition or motivation, as this oddball task included conditions that placed little demand on either domain. Oddball studies that have manipulated task relevance or performance feedback for oddball stimuli continue to find P3 impairment in ADHD regardless of motivational significance (35 , 39 , 74 , 75) . While this does not rule out the possibility that performance is globally affected in ADHD by deficits in motivation or effort, the data more likely indicate a form of executive dysfunction in attentional allocation to rare, salient stimuli that has yet to be fully detailed in neurocognitive models of ADHD.
This study examined a larger sample than most previous fMRI studies of ADHD and more rigorously controlled sampling and clinical characteristics than many oddball ERP studies. Despite these strengths, our analysis does not directly link the ADHD hemodynamic deficits to ERPs, which would bolster the functional interpretation of these results. Several brain regions found here to be abnormal in ADHD have been linked to other ERPs, such as the N1 (17 , 19 , 34 , 76 – 78) or N2 ERPs (34) . Future work fusing ERP/fMRI work would extend our findings in this study and help localize hemodynamic dysfunction in ADHD associated with specific attention-related cognitive processes.
Finally, we reported some group differences supported by evidence from relatively low statistical thresholds, despite being consistent with a priori hypotheses. Additional work is needed to replicate ADHD abnormalities in these brain regions.
In summary, this study links dysfunction in several specific brain regions to attention and working memory disruption in adolescents with ADHD, consistent with proposed dopamine hypofunction models of ADHD pathophysiology. In the context of the current strong theoretical and empirical focus on response inhibition or reward system deficits in ADHD, this study is important because it emphasizes that the attentional impairments in ADHD also have a distinct neurobiological basis. The cortical focus of these attention-related brain function abnormalities suggests that they may be separable from the proposed ADHD response inhibition or reward system dysfunction that more strongly implicates striatum dysfunction. This adds more evidence to proposals that the causes of ADHD are multifactorial, involving different influences on the same general neurobiological system.
1. American Psychiatric Association: Diagnostic and Statistical Manual of Mental Disorders, 4th ed. Washington, DC, American Psychiatric Association, 1994Google Scholar
2. Pennington BF, Ozonoff S: Executive functions and developmental psychopathology. J Child Psychol Psychiatry 1996; 37:51–87Google Scholar
3. Willcutt EG, Doyle AE, Nigg JT, Faraone SV, Pennington BF: Validity of the executive function theory of attention-deficit/hyperactivity disorder: a meta-analytic review. Biol Psychiatry 2005; 57:1336–1346Google Scholar
4. Barry RJ, Johnstone SJ, Clarke AR: A review of electrophysiology in attention-deficit/hyperactivity disorder, II: event-related potentials. Clin Neurophysiol 2003; 114:184–198Google Scholar
5. Bush G, Valera EM, Seidman LJ: Functional neuroimaging of attention-deficit/hyperactivity disorder: a review and suggested future directions. Biol Psychiatry 2005; 57:1273–1284Google Scholar
6. Castellanos FX: Neuroimaging studies of ADHD, in Stimulant Drugs and ADHD: Basic and Clinical Neuroscience. Edited by Solanto MV, Arnsten AFT, Castellanos FX. New York, Oxford University Press, 2001, pp 243–258Google Scholar
7. Faraone SV, Perlis RH, Doyle AE, Smoller JW, Goralnick JJ, Holmgren MA, Sklar P: Molecular genetics of attention-deficit/hyperactivity disorder. Biol Psychiatry 2005; 57:1313–1323Google Scholar
8. Swanson JM, Casey BJ, Nigg JT, Castellanos FX, Volkow ND, Taylor E: Clinical and cognitive definitions of attention deficits in children with attention-deficit/hyperactivity disorder, in Cognitive Neuroscience of Attention. Edited by Posner MI. New York, Guilford, 2004, pp 477–458Google Scholar
9. Nigg JT, Willcutt EG, Doyle AE, Sonuga-Barke EJ: Causal heterogeneity in attention-deficit/hyperactivity disorder: do we need neuropsychologically impaired subtypes? Biol Psychiatry 2005; 57:1224–1230Google Scholar
10. Hillyard SA, Picton TW: Electrophysiology of cognition, in Handbook of Physiology, section I: The nervous system, vol V: Higher Functions of the Brain. Edited by Montcastle VB, Plum F, Geiger SR. Bethesda, Md, American Physiological Society, 1987, pp 519–584Google Scholar
11. Halgren E, Marinkovic K, Chauvel P: Generators of the late cognitive potentials in auditory and visual oddball tasks. Electroencephalogr Clin Neurophysiol 1998; 106:156–164Google Scholar
12. Courchesne E, Hillyard SA, Galambos R: Stimulus novelty, task relevance, and the visual evoked potential in man. Electroencephalogr Clin Neurophysiol 1975; 39:131–143Google Scholar
13. Spencer KM, Dien J, Donchin E: Spatiotemporal analysis of the late ERP responses to deviant stimuli. Psychophysiology 2001; 38:343–358Google Scholar
14. Spencer KM, Dien J, Donchin E: A componential analysis of the ERP elicited by novel events using a dense electrode array. Psychophysiology 1999; 36:409–414Google Scholar
15. Sutton S, Baren M, Zubin J, John ER: Evoked potential correlates of stimulus uncertainty. Science 1965; 150:1187–1188Google Scholar
16. Donchin E, Coles MGH: Is the P300 component a manifestation of context updating? Behav Brain Sci 1988; 11:357–374Google Scholar
17. Halgren E, Baudena P, Clarke JM, Heit G, Liégeois C, Chauvel P, Musolino A: Intracerebral potentials to rare target and distractor auditory and visual stimuli, I: superior temporal plane and parietal lobe. Electroencephalogr Clin Neurophysiol 1995; 94:191–220Google Scholar
18. Baudena P, Halgren E, Heit G, Clarke JM: Intracerebral potentials to rare target and distractor auditory and visual stimuli, III: frontal cortex. Electroencephalogr Clin Neurophysiol 1995; 94:251–264Google Scholar
19. Halgren E, Baudena P, Clarke JM, Heit G, Marinkovic K, Devaux B, Vignal JP, Biraben A: Intracerebral potentials to rare target and distractor auditory and visual stimuli, II: medial, lateral, and posterior temporal lobe. Electroencephalogr Clin Neurophysiol 1995; 94:229–250Google Scholar
20. Casey BJ, Forman SD, Franzen P, Berkowitz A, Braver TS, Nystrom LE, Thomas KM, Noll DC: Sensitivity of prefrontal cortex to changes in target probability: a functional MRI study. Hum Brain Mapp 2001; 13:26–33Google Scholar
21. Clark VP, Fannon S, Lai S, Benson R, Bauer L: Responses to rare visual target and distractor stimuli using event-related fMRI. J Neurophysiol 2000; 83:3133–3139Google Scholar
22. Clark VP, Fannon S, Lai S, Benson R: Paradigm-dependent modulation of event-related fMRI activity evoked by the oddball task. Hum Brain Mapp 2001; 14:116–127Google Scholar
23. Horovitz SG, Skudlarski P, Gore JC: Correlations and dissociations between BOLD signal and P300 amplitude in an auditory oddball task: a parametric approach to combining fMRI and ERP. Magn Reson Imaging 2002; 20:319–325Google Scholar
24. Kiehl KA, Laurens KR, Duty TL, Forster BB, Liddle PF: Neural sources involved in auditory target detection and novelty processing: an event-related fMRI study. Psychophysiology 2001; 38:133–142Google Scholar
25. Kiehl KA, Laurens KR, Duty TL, Forster BB, Liddle PF: An event-related fMRI study of visual and auditory oddball tasks. Psychophysiology 2001; 21:221–240Google Scholar
26. Kiehl KA, Stevens MC, Laurens KR, Pearlson G, Calhoun VD, Liddle PF: An adaptive reflexive processing model of neurocognitive function: supporting evidence from a large scale (N=100) fMRI study of an auditory oddball task. Neuroimage 2005; 25:899–915Google Scholar
27. Kirino E, Belger A, Goldman-Rakic P, McCarthy G: Prefrontal activation evoked by infrequent target and novel stimuli in a visual target detection task: an event-related functional magnetic resonance imaging study. J Neuroscience 2000; 20:6612–6618Google Scholar
28. Liddle PF, Laurens KR, Kiehl KA, Ngan ETC: Abnormal function of the brain system supporting motivated attention in medicated patients with schizophrenia: an fMRI study. Psychol Med 2006; 36:1097–1108Google Scholar
29. Linden DE, Prvulovic D, Formisano E, Voellinger M, Zanella FE, Goebel R, Dierks T: The functional neuroanatomy of target detection: an fMRI study of visual and auditory oddball tasks. Cerebral Cortex 1999; 9:815–823Google Scholar
30. McCarthy G, Luby M, Gore J, Goldman-Rakic P: Infrequent events transiently activate human prefrontal and parietal cortex as measured by functional MRI. J Neurophysiology 1997; 77:1630–1634Google Scholar
31. Menon V, Ford JM, Lim KO, Glover GH, Pfefferbaum A: Combined event-related fMRI and EEG evidence for temporal-parietal cortex activation during target detection. Neuroreport 1997; 8:3029–3037Google Scholar
32. Mulert C, Jager L, Schmitt R, Bussfeld P, Pogarell O, Moller HJ, Juckel G, Hegerl U: Integration of fMRI and simultaneous EEG: towards a comprehensive understanding of localization and time-course of brain activity in target detection. Neuroimage 2004; 22:83–94Google Scholar
33. Opitz B, Mecklinger A, Von Cramon DY, Kruggel F: Combining electrophysiological and hemodynamic measures of the auditory oddball. Psychophysiology 1999; 36:142–147Google Scholar
34. Calhoun VD, Adali T, Pearlson GD, Kiehl KA: Neuronal chronometry of target detection: fusion of hemodynamic and event-related potential data. Neuroimage 2006; 30:544–553Google Scholar
35. Holcomb PJ, Ackerman PT, Dykman RA: Auditory event-related potentials in attention and reading disabled boys. Int J Psychophysiol 1986; 3:263–273Google Scholar
36. Jonkman LM, Kemner C, Verbaten MN, Koelega HS, Camfferman G, vd Gaag RJ, Buitelaar JK, van Engeland H: Event-related potentials and performance of attention-deficit hyperactivity disorder: children and normal controls in auditory and visual selective attention tasks. Biol Psychiatry 1997; 41:595–611Google Scholar
37. Johnstone SJ, Barry RJ, Anderson JW: Topographic distribution and developmental timecourse of auditory event-related potentials in two subtypes of attention-deficit hyperactivity disorder. Int J Psychophysiol 2001; 42:73–94Google Scholar
38. Johnstone SJ, Barry RJ: Auditory event-related potentials to a two-tone discrimination paradigm in attention deficit hyperactivity disorder. Psychiatry Res 1996; 64:179–192Google Scholar
39. Kemner C, Verbaten MN, Koelega HS, Buitelaar JK, van der Gaag RJ, Camfferman G, van Engeland H: Event-related brain potentials in children with attention-deficit and hyperactivity disorder: effects of stimulus deviancy and task relevance in the visual and auditory modality. Biol Psychiatry 1996; 40:522–534Google Scholar
40. Kuperman S, Johnson B, Arndt S, Lindgren S, Wolraich M: Quantitative EEG differences in a nonclinical sample of children with ADHD and undifferentiated ADD. J Am Acad Child Adolesc Psychiatry 1996; 35:1009–1017Google Scholar
41. Ozdag MF, Yorbik O, Ulas UH, Hamamcioglu K, Vural O: Effect of methylphenidate on auditory event related potential in boys with attention deficit hyperactivity disorder. Int J Pediatr Otorhinolaryngol 2004; 68:1267–1272Google Scholar
42. Hermens DF, Williams LM, Clarke S, Kohn M, Cooper N, Gordon E: Responses to methylphenidate in adolescent AD/HD: evidence from concurrently recorded autonomic (EDA) and central (EEG and ERP) measures. Int J Psychophysiol 2005; 58:21–33Google Scholar
43. Lazzaro I, Anderson J, Gordon E, Clarke S, Leong J, Meares R: Single trial variability within the P300 (250–500 ms) processing window in adolescents with attention deficit hyperactivity disorder. Psychiatry Res 73:91–101, 1997Google Scholar
44. Lazzaro I, Gordon E, Whitmont S, Meares R, Clarke S: The modulation of late component event related potentials by pre-stimulus EEG theta activity in ADHD. Int J Neurosci 107:247–264, 2001Google Scholar
45. Tamm L, Menon V, Reiss AL: Parietal attentional system aberrations during target detection in adolescents with attention deficit hyperactivity disorder: event-related fMRI evidence. Am J Psychiatry 2006; 163:1033–1043Google Scholar
46. Bush G, Frazier JA, Rauch SL, Seidman LJ, Whale PJ, Jenike MA, Rosen BR, Biederman J: Anterior cingulate cortex dysfunction in attention-deficit/hyperactivity disorder revealed by fMRI and the counting stroop. Biol Psychiatry 1999; 45:1542–1552Google Scholar
47. Durston S, Tottenham NT, Thomas KM, Davidson MC, Eigsti IM, Yang Y, Ulug AM, Casey BJ: Differential patterns of striatal activation in young children with and without ADHD. Biol Psychiatry 2003; 53:871–878Google Scholar
48. Rubia K, Overmeyer S, Taylor E, Brammer M, Williams SCR, Simmons A, Bullmore ET: Hypofrontality in attention deficit hyperactivity disorder during higher-order motor control: a study with functional MRI. Am J Psychiatry 1999; 156:891–896Google Scholar
49. Tamm L, Menon V, Ringel J, Reiss AL: Event-related fMRI evidence of frontotemporal involvement in aberrant response inhibition and task switching in attention-deficit/hyperactivity disorder. J Am Acad Child Adolesc Psychiatry 2004; 43:1430–1440Google Scholar
50. Rubia K, Smith AB, Brammer MJ, Toone B, Taylor E: Abnormal brain activation during inhibition and error detection in medication-naive adolescents with ADHD. Am J Psychiatry 2005; 162:1067–1075Google Scholar
51. Kaufman J, Birmaher B, Brent D, Rao U, Flynn C, Moreci P, Williamson D, Ryan N: Schedule for Affective Disorders and Schizophrenia for School-Age Children—Present and Lifetime Version (K-SADS-PL): initial reliability and validity data. J Am Acad Child Adolesc Psychiatry 1997; 36:980–988Google Scholar
52. Hollingshead ADB, Redlich FC: Social Class and Mental Illness: A Community Study. New York, John Wiley & Sons, 1958Google Scholar
53. Beck AT, Steer RA, Brown GK: Beck Depression Inventory–II (BDI–II). San Antonio, Tex, Harcourt Assessment, 1996Google Scholar
54. March JS, Parker JD, Sullivan K, Stallings P, Conners CK: The Multidimensional Anxiety Scale for Children (MASC): factor structure, reliability, and validity. J Am Acad Child Adolesc Psychiatry 1997; 36:554–565Google Scholar
55. Wilkinson GS: Wide Range Achievement Test. Wilmington, Del, Wide Range, 1993Google Scholar
56. Conners CK: Conners’ Continuous Performance Test II. Lutz, FL, Psychological Assessment Resources, 2005Google Scholar
57. Stevens MC, Calhoun VD, Kiehl KA: Hemispheric differences in hemodynamics elicited by auditory oddball stimuli. Neuroimage 2005; 26:782–792Google Scholar
58. Stevens MC, Calhoun VD, Kiehl KA: fMRI in an oddball task: effects of target-to-target interval. Psychophysiology 2005; 42:636–642Google Scholar
59. Freire L, Mangin JF: Motion correction algorithms may create spurious brain activations in the absence of subject motion. Neuroimage 2001; 14:709–722Google Scholar
60. Freire L, Roche A, Mangin JF: What is the best similarity measure for motion correction in fMRI time series? IEEE Trans Med Imaging 2002; 21:470–484Google Scholar
61. Josephs O, Henson RN: Event-related functional magnetic resonance imaging: modelling, inference, and optimization. Philos Trans R Soc Lond B Biol Sci 1999; 354:1215–1228Google Scholar
62. Calhoun VD, Stevens MC, Pearlson GD, Kiehl KA: fMRI analysis with the general linear model: removal of latency-induced amplitude bias by incorporation of hemodynamic derivative terms. Neuroimage 2004; 22:252–257Google Scholar
63. Van Essen DC, Drury HA, Dickson J, Harwell J, Hanlon D, Anderson CH: An integrated software suite for surface-based analyses of cerebral cortex. J Am Med Inform Assoc 2001; 8:443–459Google Scholar
64. Nigg JT, Casey BJ: An integrative theory of attention-deficit/hyperactivity disorder based on the cognitive and affective neurosciences. Dev Psychopathol 2005; 17:785–806Google Scholar
65. Volkow ND, Wang GJ, Fowler JS, Ding YS: Imaging the effects of methylphenidate on brain dopamine: new model on its therapeutic actions for attention-deficit/hyperactivity disorder. Biol Psychiatry 2005; 57:1410–1415Google Scholar
66. Nigg JT, Stavro G, Ettenhofer M, Hambrick DZ, Miller T, Henderson JM: Executive functions and ADHD in adults: evidence for selective effects on ADHD symptom domains. J Abnorm Psychol 2005; 114:706–717Google Scholar
67. Castellanos FX, Sonuga-Barke EJ, Milham MP, Tannock R: Characterizing cognition in ADHD: beyond executive dysfunction. Trends Cogn Sci 2006; 10:117–123Google Scholar
68. Desjardins AE, Kiehl KA, Liddle PF: Removal of confounding effects of global signal in functional MRI analyses. Neuroimage 2001; 13:751–758Google Scholar
69. Sonuga-Barke EJ: Causal models of attention-deficit/hyperactivity disorder: from common simple deficits to multiple developmental pathways. Biol Psychiatry 2005; 57:1231–1238Google Scholar
70. Pennington BF: Toward a new neuropsychological model of attention-deficit/hyperactivity disorder: subtypes and multiple deficits. Biol Psychiatry 2005; 57:1221–1223Google Scholar
71. Aron AR, Poldrack RA: The cognitive neuroscience of response inhibition: relevance for genetic research in attention-deficit/hyperactivity disorder. Biol Psychiatry 2005; 57:1285–1292Google Scholar
72. Barkley RA: ADHD and the Nature of Self-Control. New York, Guilford, 1997Google Scholar
73. Sergeant JA: Modeling attention-deficit/hyperactivity disorder: a critical appraisal of the cognitive-energetic model. Biol Psychiatry 2005; 57:1248–1255Google Scholar
74. Holcomb PJ, Ackerman PT, Dykman RA: Cognitive event-related brain potentials in children with attention and reading deficits. Psychophysiology 1985; 22:656–667Google Scholar
75. Satterfield JH, Schell AM, Nicholas TW, Satterfield BT, Freese TE: Ontogeny of selective attention effects on event-related potentials in attention-deficit hyperactivity disorder and normal boys. Biol Psychiatry 1990; 28:879–903Google Scholar
76. Anderer P, Pascual-Marqui RD, Semlitsch HV, Saletu B: Differential effects of normal aging on sources of standard N1, target N1, and target P300 auditory event-related brain potentials revealed by low resolution electromagnetic tomography (LORETA). Electroencephalogr Clin Neurophysiol 1998; 108:160–174Google Scholar
77. Giard MH, Perrin F, Echallier JF, Thevenet M, Froment JC, Pernier J: Dissociation of temporal and frontal components in the human auditory N1 wave: a scalp current density and dipole model analysis. Electroencephalogr Clin Neurophysiol 1994; 92:238–252Google Scholar
78. Liegeois-Chauvel C, Musolino A, Badier JM, Marquis P, Chauvel P: Evoked potentials recorded from the auditory cortex in man: evaluation and topography of the middle latency components. Electroencephalogr Clin Neurophysiol 1994; 92:204–214Google Scholar